Chapter structure
- 4.1 Introduction: The Contribution of Plants to the Global Carbon Cycle
- 4.2 Is the Glass Half Full or Half Empty?
- 4.3 Low Energy Conversion Efficiency in Photosynthesis
- 4.4 The Use of Photosynthesis to Drive Plant Growth
- 4.5 Vegetative Biomass: What to Do with the Cell Walls?
- 4.6 Response of Photosynthesis and Plant Growth
to Rising Atmospheric Carbon Dioxide - 4.7 Integrated Model of Energy Use Efficiency
during Photosynthesis and Growth - 4.8 What is Needed for Efficient Energy Crops?
- 4.9 Current Energy Crops and Their Shortcomings
- Footnotes
4.1 Introduction: The Contribution of Plants to the Global Carbon Cycle
Plants, together with algae and photosynthetic bacteria, are the only living organisms that can use sunlight as an energy source to drive chemical reactions in a process called photosynthesis. They are the basis of all life on earth and play a central role in the global carbon and energy cycles. This chapter provides an overview of the processes involved in photosynthesis and plant growth, analyzes the efficiency of energy conversion in plants, and discusses the consequences for the use of plant biomass as a source of bioenergy.
Biological life depends on the synthesis of myriads of molecules and macromolecules, their organization on many spatial levels to form molecular machines, cells, organs and organisms, the maintenance thereof in the face of a changing environment, and their reproduction. However, human beings and all other animals and most microbes cheat. We gain precursor molecules like sugars, lipids and amino acids by eating other organisms, and we obtain the energy necessary to drive these interconversions by degrading these compounds. Reducing groups that are extracted during their degradation through a series of coupled redox reactions, with oxygen acting as the final acceptor (Figure 4.1A). This process is called respiration. These redox reactions occur in a membrane and release energy that is captured as an electrical gradient across the membrane. This gradient then drives the synthesis of adenosine triphosphate (ATP) from adenosine diphosphate (ADP) and phosphate. ATP is a universal energy carrier that is used to drive a huge variety of biochemical reactions. But where do all the complex molecules in the organisms we eat come from? What energy input drives the biological world?
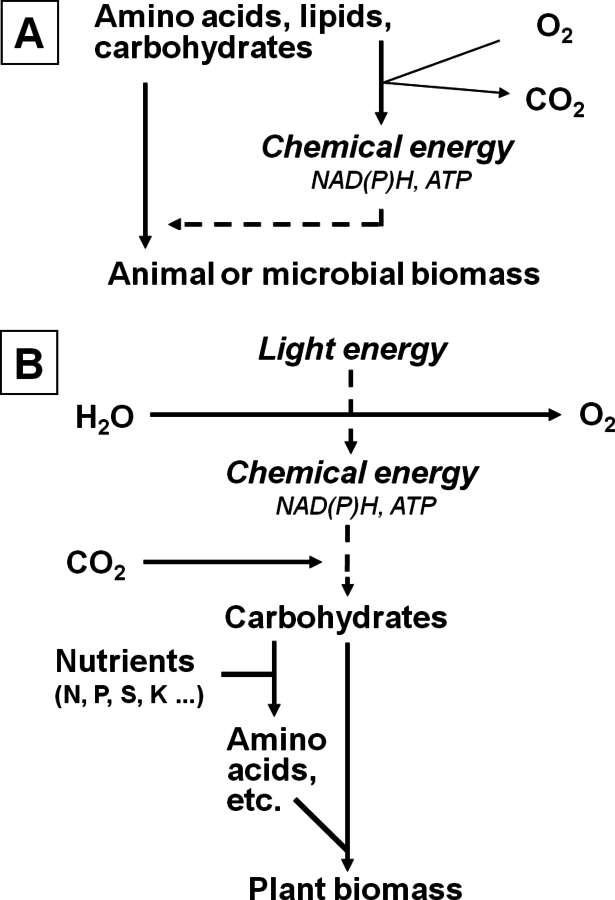
Fig. 4.1: A schematic representation of metabolism in non-photosynthetic and photosynthetic organisms. (A) Non-photosynthetic organisms—a microbe or an animal. (B) A photosynthetic organism—an algae or a plant. The flow of energy is indicated as dotted lines. For redox equivalents, this can occur via NADH (especially for use in respiration to produce ATP) or NADPH (especially to provide redox groups for biosynthesis, and in photosynthesis). For simplification, uptake of water (up to 70% of the fresh weight for microbes and animals, and 90% for plants) is omitted.
Many early forms of life used reduced inorganic compounds as a source of energy. Other organisms developed ways to use light energy to oxidize reduced inorganic compounds, thereby generating reducing groups and energy. This is still done today by many bacteria. The decisive step leading to the world as we know it today, however, was the development of what is termed oxygenic photosynthesis. In this process, light energy is used to oxidize water to oxygen and hydrogen (Figure 4.1B). This releases very electronegative reducing groups, which pass down a series of redox reactions, releasing energy that is used to make ATP. The reducing groups are ultimately used to generate a molecule called NADPH. The ATP and NADPH are used to drive a plethora of other reactions. The most important reaction is the conversion of
into carbohydrates. Other reactions include the conversion of nitrate into ammonium, or sulphate into reduced sulphur-containing molecules. The ammonium and reduced sulphur compounds are combined with molecules derived from carbohydrates to generate a multitude of N- and S-containing molecules like amino acids and nucleic acids.
Plants can be viewed as solar-powered chemical factories, which take a range of inorganic compounds (carbon dioxide, nitrate, sulphate, phosphate, iron and many other metals) and use them to produce an enormous range of exquisite organic molecules and macromolecules. Their range and precision far exceed that of chemical factories constructed by men. They do this with a minimum of environmental side effects. Not only the synthesis but also the transport of these chemicals is driven by solar power. For example, nutrients are transported from the soil to the leaves of the plants, up to a hundred meters high in the largest trees, in a flow of water (technically termed the transpiration stream) that is driven by the evaporation of water in the leaves and drawn up as a column of water through fine vessels (technically called the xylem) in the stems.
Oxygenic photosynthesis is the direct or indirect source of almost all life on earth as we see it today. It is the direct source of energy and biological molecules for the growth of plants. It is the indirect source of energy and biological molecules for most bacteria and all fungi and animals. These either feed on plants, or live by degrading dead plant matter, or by eating animals that themselves live off plants.
Oxygenic photosynthesis is also a dominating factor in the global carbon and oxygen cycles that determine the composition of the atmosphere. Briefly, plants use light energy from the atmosphere to assimilate carbon dioxide into reduced organic molecules, thereby releasing oxygen. The plants are themselves eaten by animals, leading to an uptake of oxygen and a release of carbon dioxide. Alternatively, when plants die they decay due to the activity of bacteria, fungi or animals, again leading to an uptake of oxygen and a release of carbon dioxide. Some of the carbon in plants is sequestered in the soil, either because the plant matter is not degraded, or because it accumulates in residues from the organisms that eat plants or degraded dead plant matter. In the short term, this is an important interim pool of carbon whose disturbance can lead to a sudden release of carbon dioxide into the atmosphere. For example, this occurs when a forest is cleared. In the most extreme case, when plant matter is not degraded, large amounts of organic carbon can accumulate and, over millions of years, be converted into highly reduced forms like coal, oil or gas.
For thousands of years, mankind obtained most of its energy from living or newly dead plant material by burning wood, or by eating plants or animals to provide energy to fuel the muscular system. For some specific tasks, also water (e.g., water wheel driven corn mills) or wind (sailing ships, wind mills) were used. It might be noted that by the 18th century, there was a large-scale use of wood for charcoal production. There were also perceived worries about the lack of wood for other uses such as the building of ships (Dudlay Pope, “Life in Nelson’s Navy”1). This serves as a good example for the potential conflict between the use of plant biomass as a source of material versus its use as a source of energy, which will be discussed later in this chapter and also in other chapters of this book. Starting with the industrial revolution in England in the 18th century, and then accelerating with time and spreading over the entire globe, coal, and later oil and gas, were used as energy sources with a still increasing use. They are often termed fossil fuels because they are derived from long-dead plant matter.
By the 1970s there were worries that the finite resources of fossil fuels would become exhausted (see “The Club of Rome”). These worries probably underestimated the amount of undiscovered fossil fuels and the extent to which improved technology and increasing prices for these fuels would allow known but previously inaccessible sources to be used. Research in the last decades, which has led to an increased understanding of the global carbon cycle and the role of carbon dioxide as a greenhouse gas, has led to even more pressing reasons to decrease the use of fossil fuels. Coal, petrol and oil have been accumulating on earth for the last 350 million years. This process is now being reversed at an incredible speed. The amount of carbon dioxide that is currently being released into the atmosphere in a decade is equivalent to the amount of carbon that was sequestered underground over many millions of years. This release is one of the drivers of the present increase in global temperature. It is predicted that if the release of carbon dioxide from fossil fuels continues to increase, there will be a major global change of climate in the coming century. There is an urgent need to radically increase the use of alternative energy sources like wind power, solar power and water power.
Looked at from the point of view of the plant sciences, two questions are pinpointed: First, can bioenergy make a significant contribution to energy production? We might note that this not only requires that living biomass is converted into compounds that can be conveniently used as a source of energy, but also that this is done at a rate that is an order of a million-fold higher than occurred by geological processes in the last 350 million years. Second, can plants be used to reverse or ameliorate the increase in atmospheric
?
4.2 Is the Glass Half Full or Half Empty?
Let us start off by being optimistic. More solar energy reaches the earth’s surface every hour (4.3 x 1012J) than is consumed by humans in a year (4.1 x 1012J ) (Basic Research Needs for Solar Energy Utilization, U.S. Department of Energy Solar Energy Workshop Report2). Plants have been performing photosynthesis for hundreds of millions of years. We might therefore expect them to have become highly optimized for conversion of this solar energy into biomass, which can then be used to generate energy. Further, the
concentration found in the atmosphere actually limits photosynthesis in most plants. This simple fact can be demonstrated by monitoring the rate of photosynthesis and suddenly increasing the carbon dioxide concentration. It is exploited in the commercial greenhouse industry, which often uses elevated carbon dioxide concentrations to speed up plant growth. Thus, the current increase in atmospheric carbon dioxide concentrations would be expected to allow more photosynthesis globally, and result in an increase in biomass formation and crop yield on agriculturally-used land. Will there be a large enough surplus to provide feedstocks for the large-scale production of bioenergy?
To assess this hope, let us look at the current situation with respect to food production. If there is a large amount of “low hanging fruit” to be used for bioenergy production, we would surely expect there to be a large surplus in food production. This is patently not the case. The following assessment is based on a global perspective and does not address local cases where there is a local surplus, or at least a perceived surplus, as a result of very favorable local terrain and climatic conditions, large financial subsidies, having the wealth to import food, or other economic or political factors. First, despite large increases in crop yield in the last half century as a result of the green revolution, the current global agricultural production is scarcely able to keep pace with the growing demand for food as the world population grows and the standards of living in many parts of the world slowly improve [1]. Second, it is unlikely that more land can be used for agricultural production, neither for food nor for energy purposes [2]. Much of the land that is not currently used has unsuitable terrain or climatic conditions. Other large areas that are not used for agriculture support large scale ecosystems that themselves play a key role in the global carbon and water cycles, such as the tropical rainforests, whose deforestation would lead to the release of so much sequestered carbon that its effect could not be counterbalanced in decades. Third, there are signs that the yield gain is levelling off [3-7].
In the future it will be necessary to find new ways to increase crop yields just to keep pace with the growing global demand for food. The yield increases of the green revolution were achieved by advances in plant breeding and the increased use of water, fertilizers and pesticides. It is thought that these contributed approximately equally to the yield gain, although the contribution may have varied depending on the type of crop and geographical region [3,6,8]. In the future, it will be desirable to decrease the use of fertilizers, water and pesticides. Widespread use of fertilizers and pesticides has a significant negative impact on the environment [9,10]. There are finite reserves of some fertilizers (e.g., phosphates), and the production of others (especially nitrogen fertilizers via the Haber-Bosch process) consumes large amounts of energy. In the context of using bioenergy to decrease the use of fossil fuels and
release to the atmosphere, this is a serious drawback! Irrigation can lead to a gradual loss of soil quality due to salinisation and contamination with other chemicals. Besides, in areas where irrigation is necessary and widespread, water is often a rare resource and tends to become even more scarce. Agriculture accounts for over 70% of the total water use in most developed countries (World Agriculture: Towards 2015/2030, FAO, 20023).
This rather disturbing analysis leads to several conclusions with respect to any use of plants as a large-scale source of bioenergy, or as a quantitatively important feedstock for the chemical industry. First, it will be essential that production of bioenergy does not compete with food production. Second, it will be essential to maintain and even increase the yield per unit area of agricultural land, irrespective of whether the plants are going to be used for food only, or for energy and chemical feedstocks as well. Third, this will probably have to be done while decreasing the use of water, fertilizers and pesticides.
Further large advances in either agronomic practice and/or in plant breeding will be required to allow yields to be maintained at current or even higher levels, while decreasing the use of water, fertilizers and pesticides. Although agronomic practice and plant breeding are sometimes seen as alternative routes, it is most likely that it will be the successful fusion of both that will allow further increases in yield [1]. Plant breeders select and optimize crops for their performance in a given cultivation regime. A change in the cultivation regime is likely to create a situation that is not fully exploited by current cultivars, opening a new window of opportunity for plant breeding. Conversely, an advance in breeding or the production of a plant with a major improvement in an existing trait or even a new trait can open up new strategies in agronomic practice.
This interplay between breeding and agronomic practice can be illustrated by two examples from maize breeding. First, the increase in yield per hectare in the last decades has been largely achieved by breeding maize so that it can be packed more densely on the field—i.e., by a very close interaction between breeding and a simple change in agronomic practice [6]. The second example is Roundup Ready maize, a genetically modified organism. This GMO was developed to aid weed control and carries a transgene that confers tolerance to the herbicide glyphosate. However, one of the main advantages of Roundup Ready maize is that it can be sown by drill boring, rather than by ploughing. Previously, maize was sown after ploughing and treatment of the soil with herbicide to remove competing weeds before planting the maize seeds, with the concomitant use of energy for the machines and loss of soil by blow-off. Roundup Ready maize can be drill-sown in small holes bored into unploughed land, and the competing weeds are killed later by the application of glyphosate. Irrespective of the political discussion over the use of GMO crops, this example illustrates how a new trait in the crop can facilitate a change in agricultural practice.
The next sections of this chapter will outline some basic principles underlying the process of photosynthesis and plant growth, which are central to plant yield. At the start of the this section, three rather optimistic statements were made:
1Natural selection over hundreds of millions of years should have optimized the energy conversion and growth rates in plants.
2The amount of energy needed from plants is minuscule in comparison with the total irradiation intercepted by the earth.
3Rising atmospheric
should lead to an increase in photosynthesis and, by implication, to plant growth.
The fact that hardly enough food can be produced for mankind arouses the suspicion that there is a fly in the ointment. In fact, there are several. First, energy conversion in plants is actually inherently inefficient. Second, momentary increases in the rate of photosynthesis often fail to translate into a sustained increase in growth and biomass formation. Third, much of the plant biomass that is produced, even on agricultural fields and even less so in semi-natural or natural ecosystems, cannot be used as food—at least for human consumption—and is also not usable for bioenergy, at least with current technologies. To understand why this is so, we need to look more closely at the processes involved in photosynthesis and plant growth and at the function and composition of different parts of the plant.
4.3 Low Energy Conversion Efficiency in Photosynthesis
The energy conversion efficiency of photosynthesis has been the subject of two recent meta-analyses [11,12], which are summarized in the following section. Although the earth intercepts massive amounts of solar irradiation (120,000 TW per year), several factors mean that only a minute fraction of this energy is actually converted into plant biomass. Before explaining how and why the light energy is used so wastefully, I will first briefly introduce the pathway of photosynthesis.
4.3.1 Photosynthesis
Figure 4.2 provides a schematic overview of the energy and carbon flow during photosynthesis. This is often divided into light reactions and dark reactions (for a simple introduction, see also Michel [13].
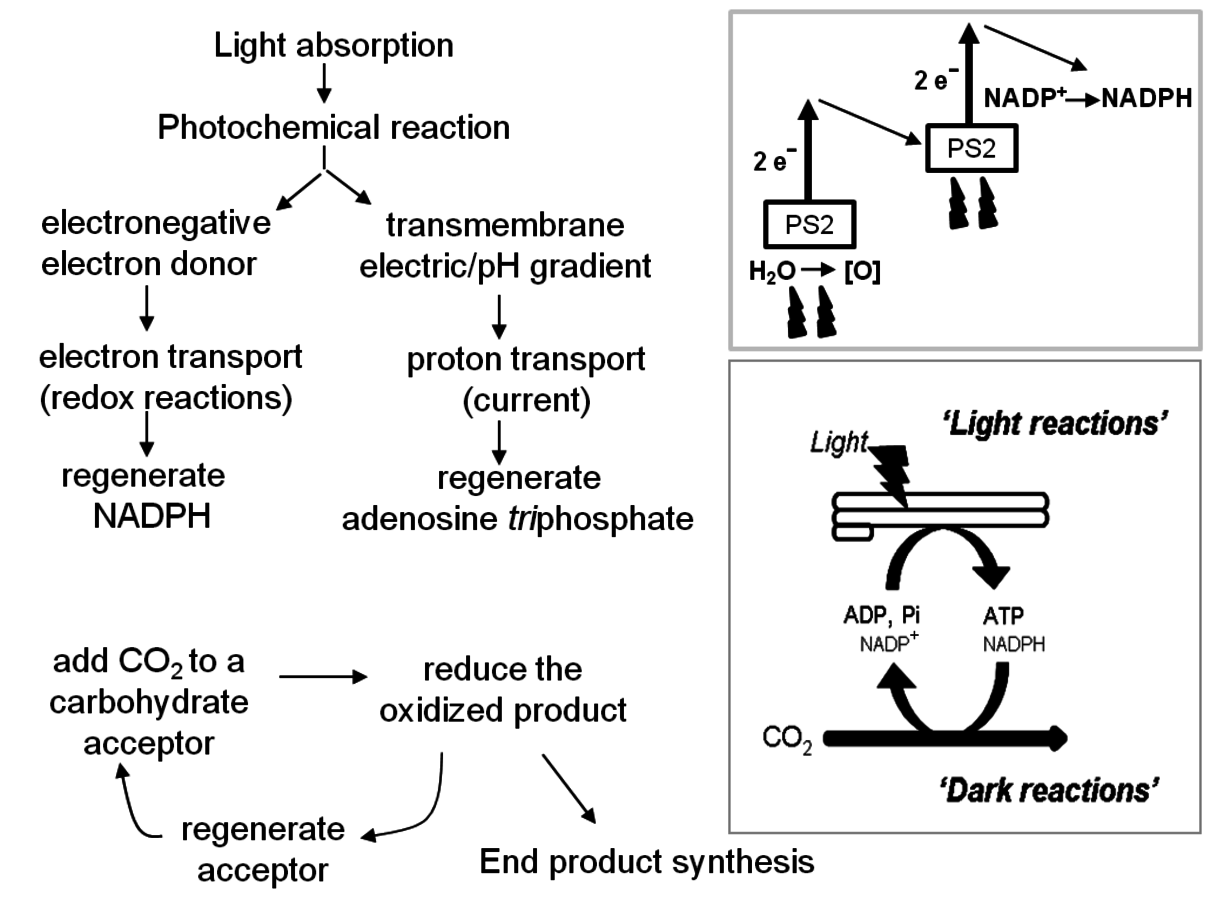
Fig. 4.2: A schematic representation of photosynthesis. The lower insert shows the conceptual separation of the light reactions in the chloroplast thylakoid membranes and the dark reactions in the soluble phase of the chloroplast. The main panel summarizes the main sub-processes in the light reactions and in the dark reactions. The upper level insert indicates the two sites (photochemical reactions) at which light energy is used to drive the light reactions.
The first step in the light reactions is the absorption of light by chlorophyll. This leads to the excitation of an electron, which moves into a higher orbital. The energy is transferred across an array of chlorophyll molecules to the so-called reaction center. In the reaction center the energized electron drives a redox reaction. There are actually two reaction centers, arranged in series, in oxygenic photosynthesis. Starting with water, the first photochemical reaction (confusingly termed Photosystem II), splits water to release oxygen and hydrogen. The reducing groups of the hydrogen then pass through a series of redox reactions and are ultimately used to reduce
to NADPH. At one step during this process, the reducing groups are transferred up to a more electronegative acceptor, using energy from the second light-driven reaction (termed Photosystem I). Energy that is released during this chain of reactions is stored as an electrical or pH gradient across a membrane in which the light reactions occur. The electrical or pH gradient is used to convert ADP and orthophosphate into ATP. Two light-driven reactions in series are needed to provide the energy to drive movement of a reducing equivalent from water to NADPH and at the same time to generate ATP.
NADPH and ATP are used in the dark reactions to drive the conversion of
into carbohydrates. The first step in the dark reactions is a unique reaction found only in photosynthetic organisms, in which a 5-carbon high-energy acceptor molecule (ribulose-1,5-bisphosphate) reacts with carbon dioxide to form two molecules of glycerate-3-phosphate. This reaction is catalyzed by an enzyme called ribulose-1,5-bisphosphate carboxylase/oxygenase (abbreviated RubisCO; see later for why it has the additional oxygenase in its name). Glycerate-3-phosphate is then reduced to triose-phosphate (triose denotes a carbohydrate with 3-carbons). The reduction of glycerate-3-phosphate to triose phosphate uses almost all the NADPH and most of the ATP that is generated in the light reactions. Most of the triose phosphate is used to regenerate the high energy carbon acceptor in a complex multistep pathway that requires ATP. This entire process is called the Calvin-Benson cycle, after the scientists who discovered it (see [14,15] for historical reviews). The net gain in carbon can be converted to end products like sucrose and starch. Sucrose is exported to the rest of the plant to support metabolism and growth, while starch is stored during the day and later remobilized at night to support metabolism and growth at night [16].
So where is energy wasted in this process? The rate of plant growth can be modelled as the amount of irradiation intercepted multiplied by the efficiency with which energy in the irradiation is converted into plant matter. The very low global efficiency in the use of solar energy has two reasons; only a small part of the sunlight is actually intercepted by plants or other photosynthetic organisms, and the energy in the intercepted light is not efficiently used to drive carbon dioxide assimilation or (see later sections) plant growth.
4.3.2 Much of the Solar Irradiation is Not Intercepted by Plants
Over half of the total amount of sunlight falls on the oceanic regions of the earth. Although high rates of algal growth are possible in localized areas, much of the ocean is very nutrient-deficient. Nevertheless, it is estimated that about half of the primary photosynthetic biomass production is produced in the oceans [17]. The problem with the use of marine biomass for food and bioenergy is that it is the difficult harvesting because almost all of the photosynthetic organisms are unicellular algae. Of course, marine animals grazing on the algae, or on each other, have provided an important source of food and (e.g., whale blubber) energy for millennia. However, this vital energy conversion chain is threatened due to overfishing.
Turning to the solar irradiation that falls on terra firma, a large proportion hits regions without vegetation. This includes areas that are so cold or dry or mountainous or where the soil is so nutrient-poor that plant growth is impossible or very slow at best. Even on land areas that are suitable for plant growth, some of the irradiation arrives during the period of the year that is unsuitable for rapid plant growth. This would include winter in higher latitudes and mountains, and the dry season in Mediterranean-type climate zones and in areas of the subtropics and tropics that experience wet and dry seasons.
Even when climatic and edaphic (soil) conditions are favorable for plant growth, much of the sunlight may not be intercepted by plants because they have not yet developed enough leaves to fully cover the ground area (termed “closing the canopy”). This is especially striking with many crop plants in temperate zones, which are sown early in the year but take almost until mid-summer to grow enough to allow for canopy closure. As a result, the breeding of crops that can be planted early in the year or that can grow under more marginal conditions is one of the most important goals in the breeding of food crops. Developing energy crops that maximize light interception throughout the year or which can be grown in regions with only marginal conditions for the growth of food crops will also be a vital issue if bioenergy is to make a larger contribution to the global energy mix.
As vegetation becomes increasingly dense, it will start to shade itself with the result that an increasing proportion of biomass is respiring rather than photosynthesizing. This will decrease biomass gain and crop yield. In most natural vegetation systems, some large or tall species take—at least visually—a dominating role and absorb the bulk of the incident radiation, but many others have evolved to occupy niches. Some species have adapted to growing in low light. Others grow early in the year (often from underground bulbs or rhizomes) and flower and complete their life cycle before they become shadowed by the emerging leaves of the trees. In a cereal or maize field, plants start to shade themselves once canopy closure has occurred. Morphological features affecting leaf shape and angle allowing better light penetration into the canopy and decreasing or delaying self-shading in a closely packed stand of plants [18] belong among the most important traits selected by plant breeders in the last century.
4.3.3 Much of the Intercepted Irradiation Cannot be Used to
Drive Photosynthesis
Solar irradiation is emitted across a wide wavelength range from under 300 nm (UV) through visible light (390–750 nm) to infrared irradiation (up to about 2500 nm). The absorption spectrum of chlorophyll lies between about 360 and 700 nm (there are small variations, depending on the precise structure of the chlorophyll). Irradiance outside this range is not used for photosynthesis, equivalent to a “wastage” of up to half of the incident light energy [11,13]. Plants have ancillary pigments that can absorb light and transfer its energy to chlorophyll. Some plants and algae that live in water, which preferentially absorbs shorter wavelength irradiation, have modified chlorophyll molecules that slightly extend the absorption spectrum into the infrared region—this can be seen, for example, in the red algae that live near or below the low tide mark. However, there is a limit to which the absorption spectrum of the light absorption pigments can be extended into the infrared. As the light wavelength increases, the energy per photon decreases. At wavelengths above about 700 nm, light (or more precisely, light quanta) does not contain enough energy to drive photosynthetic reactions (see below for more discussion).
Another major inefficiency in energy conversion is the fact that all of the absorbed photons are used to drive the same reaction, irrespective of their wavelength [11,13]. Blue light at 380 nm contains almost twice as much energy per photon as red light at 700 nm (3.09 and 1.76 eV mol-1, respectively). Absorption of a photon at 380 nm leads to the electron being transferred to a higher orbital but it falls back immediately to the orbital that would be occupied by an electron after absorption of a photon at 700 nm of red light. In contrast, photovoltaic devices have absorption spectra that closely match incident solar radiation, allowing them to use not only visible but also infrared radiation (see [11]).
Why have living organisms not evolved to use the entire spectrum of solar radiation more efficiently? One reason is probably that photosynthesis evolved relatively late in the history of life, at a time when the basic biochemical machinery for energy conversion had already been established. By that time, universal energy carriers like ATP and NADPH were “established.” A plethora of proteins had already evolved that catalyze reactions in which ATP and NADPH act as energy and redox donors. There had already been a “selection” of reactions whose thermodynamic properties allowed them to be driven by these molecules along with protein structures that were compatible with such reactions. Like societies, the history of biological systems places a constraint on their further development. Photosynthesis evolved via the use and modification of existing proteins and energy carriers, rather than by the de nuovo design of the entire process. Irradiation with a wavelength larger than 700 nm does not have the energy to drive the oxidation of water and the other redox reactions in photosynthetic electron transport. There is interest in the possibility that the long-wavelength irradiation might be used to drive other energetically less expensive processes, for example the synthesis of extra ATP. However, this is an ambitious project, which will require extensive research and further developments in synthetic biology.
4.3.4 Photosynthesis Involves many Sequential Reactions, some Involving Small and others Large Decreases in Free Energy
Energy and metabolic interconversions in biological systems typically occur as a sequence of reactions, each introducing a small change in the molecule. This results in an inherent loss of energy. For example, based on the energy in a photon at 700 mm, the stoichiometry of the light reactions and the free energy in ATP and NADPH, it can be calculated that only 37% of the energy in red (700 nm) light is captured as ATP and NADPH [11,13]. The value falls to 21% for blue light at 380 nm. The dark reactions lead to a similar loss of energy, with only about a third of the energy from the ATP and NADPH being captured in the final carbohydrate products of photosynthesis [12].
4.3.5 In Bright Sunlight, Much of the Energy is Dissipated as Heat
The above calculations were made for photosynthesis occurring in low light. Under these conditions, every absorbed photon leads to a reducing equivalent moving through the electron transport chain. Speaking in technical terms, the “quantum efficiency” is perfect. One oxygen is evolved and one carbon dioxide molecule is fixed for every 8–9 photons absorbed, which reflects the stoichiometry of the pathways.



Fig. 4.3: The light saturation curve of photosynthesis. The thick line shows schematically the relation between light intensity and the rate of photosynthesis. In darkness, there is net oxygen uptake (respiration). As the light intensity increases, there is initially a strictly proportional increase in the rate of photosynthesis. This reflects the fact that the “quantum yield” is almost perfect, i.e., every photon absorbed is used to drive a photochemical reaction. The slope of this initial line reflects the stoichiometry of the photosynthetic pathways, with 8–9 photons being required to evolve one
(or fix one
). As the light intensity rises further, a region is reached where the rate of photosynthesis reaches a maximum level (i.e., photosynthesis is light-saturated). All incident photons above this level are absorbed but the energy is dissipated as heat rather than being used to drive a photochemical reactions.
However, as the irradiance intensity is increased, a point is reached where the rate of photosynthesis stops to increase and levels off instead (Figure 4.3). Photosynthesis is then light-saturated. The light intensity at which photosynthesis becomes light-saturated depends on the temperature, the carbon dioxide concentration, the prehistory of the leaf and the species. In the majority of plants, it is reached well below the light intensities that are experienced on sunny summer days. This means that part of the light energy is not used. It is still absorbed by chlorophyll but, instead of being used to drive photosynthesis, it is dissipated as heat [12].
Energy dissipation occurs via a regulated mechanism, which is turned off at low light intensities and is activated when photosynthesis becomes light saturated. As discussed by Zhu et al. [12], it is important that energy dissipation is switched on and off at the correct light intensities for a given leaf and condition. If energy dissipation is turned on at light intensities that are not yet saturating, the ongoing rate of photosynthesis will be decreased. If it is switched on too late, the un-dissipated light energy results in damage to the photosynthetic apparatus, which will also lead to an inhibition of photosynthesis and requires repair of the apparatus, which is itself an energy-consuming process. This raises the questions if this and other regulatory processes are optimally regulated, if it is possible for a plant to do this across a wide range of different environmental conditions, and if this is a possible target for plant breeding.
4.3.6 An Unspecific Side Reaction of the Key Enzyme RubisCO Leads to a Large Wastage of Energy, Nitrogen and Water
One of the largest sources of inefficiency in photosynthesis revolves around the enzyme RubisCO (Figure 4.4). As already mentioned, RubisCO catalyzes the key reaction in the Calvin-Benson cycle in which the acceptor ribulose-1,5-bisphos-
phate reacts with carbon dioxide to form two molecules of glycerate-3-phosphate. However, RubisCO has several side reactions, including one in which ribulose-1,5-bisphosphate reacts with oxygen instead of carbon dioxide, resulting in the formation of one molecule of glycerate-3-phosphate and one molecule of 2-phospho-
glycolate [19,20]. Oxygen is a competing substrate to carbon dioxide; as the oxygen concentration increases, the rate of the reaction with oxygen will rise and the rate of the reaction with carbon dioxide will fall. Under current atmospheric conditions (21% oxygen, 78 % nitrogen, 0.038% carbon dioxide), every third reaction uses oxygen instead of carbon dioxide, resulting in the rapid formation of 2-phosphoglycolate. 2-Phosphoglycolate is recycled by a complex metabolic pathway, termed photorespiration, which leads to the release of carbon dioxide and further energy consumption [16,20,21]. The oxygenase reaction of RubisCO and the salvaging of 2-phosphoglycolate lead to a decrease in the rate of photosynthesis of approximately 20–40% and to a 40–50% decrease in the efficiency of energy conversion [12]. This can be seen in a simple experiment, in which the side reaction is suppressed by decreasing the oxygen concentration from 21 to 2%. This leads to an immediate increase in the rate of photosynthesis. The energy loss due to photorespiration increases as the temperature rises [22] because high temperatures favor the reaction of RubisCO with oxygen as compared to carbon dioxide.
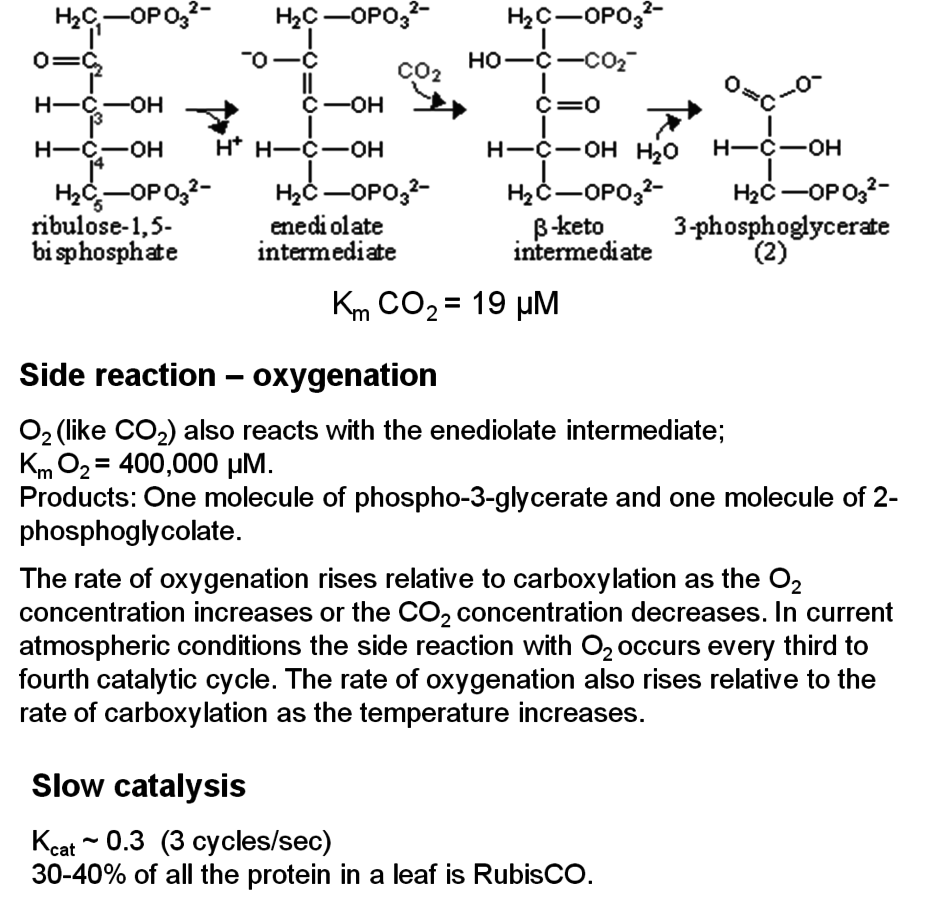
Fig. 4.4: Summary of the main features of the reactions catalyzed by ribulose-1,5-bisphosphate carboxylase/oxygenase (RubisCO). The scheme shows the reaction sequence with carbon dioxide.
Why does this crucial enzyme perform such a wasteful side reaction, and why have hundreds of millions of years of evolution not solved this “problem”? There are probably two reasons:
One relates to the catalytic mechanism of RubisCO. RubisCO is a very slow catalyst with rates of about three reactions catalyzed per second per catalytic site, compared to typical rates of 10,000 to 100,000 per second that are found for most other enzymes. There is a trade-off between the rate of catalysis and the specificity factor—the specificity for carbon dioxide relative to oxygen, such that an increased specificity for carbon dioxide results in an even lower rate of catalysis [23]. Due to its exceptionally low rate of catalysis, RubisCO already accounts for an incredible 30–40% of all proteins in a leaf. Therefore, there are strong constraints on increasing specificity at the expense of the catalytic rate.
The other reason deals with the way biological systems evolve. Oxygenic photosynthesis evolved in an atmosphere that contained high carbon dioxide and very little oxygen, under which conditions the side reaction of RubisCO with oxygen was quantitatively negligible [22]. This “construction mistake” was not revealed until the gradually falling carbon dioxide and rising oxygen concentrations in the atmosphere led to the side reaction with oxygen becoming quantitatively important. This probably did not occur until the last 400 million years. It is thought that atmospheric conditions will only have favored significant levels of photorespiration during the Carboniferous period (280–340 million years ago) and in the past 35 million years. However, by that time it was too late to change the complex dark reactions that had evolved around RubisCO and to develop an oxygen-insensitive pathway for carbon dioxide fixation instead.
Citing Sage [22]:
By the Carboniferous, all plants used RubisCO for the net carboxylation step of photosynthesis, and RubisCO was well integrated into the primary metabolism of the plant. Because of this integration, the likelihood of evolutionarily solving the photorespiratory problem within the context of C3 photosynthesis [photosynthesis using the Calvin-Benson cycle] was probably nil. Even if a novel carboxylase could be produced, it would probably be useless because the plant would lack the metabolic pathways to regenerate acceptor molecules and process the carboxylation products.
The costs of this side reaction are not limited to a lower rate of photosynthesis. As mentioned in the last paragraph, RubisCO accounts for 30–40% of total leaf protein. This has a large impact on the so-called nitrogen use efficiency of a plant—the amount of nitrogen needed to generate a given amount of biomass. This in practical terms means that crops require more nitrogen fertilizer.












Fig. 4.5: Entry of
into and loss of water from the leaf. The leaf surface is covered with a wax layer and highly impermeable to
and water vapor. However, it contains many small apertures, called stomata, whose diameter and hence conductance, g, can be changed. The fluxes of
and water vapor (
) are schematically depicted through a single stomata on the leaf epidermis. For both gases, the flux, J, depends on the concentration gradient and the stomatal conductance, g. The poor affinity of RubisCO for
and the competitive reaction with
means that
(the
concentration in the airspaces inside the leaf) must be maintained at relatively high levels. As
(the atmospheric concentration of
), is fixed, increased rates of photosynthesis and
entry can only be achieved by an increase in stomatal aperture, which increases g (stomatal conductance). This is unavoidably accompanied by an increase in the loss of water from the leaf to the atmosphere.
There is an even more important consequence with respect to water use (Figure 4.5). The leaf surface is covered by waxes, making it rather impermeable to gases and water. However, photosynthesis obviously requires entry of carbon dioxide into the plant. This occurs via small regulated apertures on the leaf surface called stomata. Stomata typically open in the light and close in the dark, when no photosynthesis occurs [12]. Carbon dioxide enters by diffusion through the stomata. The rate of entry therefore depends on the concentration gradient between the external atmosphere and the air spaces in the leaf (ΔC) and the conductance (g) of the stomata. Stomatal conductance increases when stomata open. However, other gases and water vapour will also move through the small hole that is conveniently provided by the stomata. Water moves out of the leaf, where the air spaces contain water-saturated air, into the atmosphere. Returning to RubisCO, one of the consequences of the competing side-reaction with oxygen is that a higher carbon dioxide concentration is required inside the leaf to support a given rate of photosynthesis. A higher internal carbon dioxide concentration can only be achieved by increasing g, i.e., opening the stomata further. The option to increase the external carbon dioxide is not available unless the plants are fortunate enough to be growing in a greenhouse with a supply of additional carbon dioxide! Increased opening of the stomata will, in turn, lead to an increased loss of water. In technical terms, the water use efficiency of photosynthesis is decreased, i.e., more molecules of water are evaporated per molecule of carbon dioxide fixed.
As can be imagined, there has been enormous evolutionary pressure to ameliorate or get around the self-imposed bottleneck at RubisCO. To date, no plant or any other living organism has managed to evolve a truly alternative way to carry out photosynthetic carbon dioxide fixation. However, there have been small but important gains in the specificity factor when RubisCO is compared in the evolutionarily most primitive organisms (photosynthetic bacteria) and higher plants. Equally important, various strategies have been evolved to “turn back history” and generate a high carbon dioxide concentration around RubisCO. These strategies are collectively termed carbon dioxide concentration mechanisms as illustrated in Figure 4.6. These are, in effect, “clamped on” in front of the Calvin-Benson cycle. Plants that operate the Calvin-Benson cycle without a carbon dioxide concentration mechanism are often termed “C3” plants (because the first product of carbon assimilation is the C3 compound glycerate-3-phosphate).
The carbon dioxide concentration mechanism in algae involves active transport of bicarbonate across the cell membrane. In water, carbon dioxide equilibrates with bicarbonate. Algae possess transport proteins that catalyze an energized uptake of bicarbonate from the surrounding medium into the cell. Bicarbonate then equilibrates back to carbon dioxide in the immediate spatial vicinity of RubisCO, which is localized in highly specialized structures (termed carboxysomes in photosynthetic bacteria, and pyrenoids in algae). These subcellular structures may facilitate the concentration of carbon dioxide by decreasing its back diffusion. Their molecular components and organization is still poorly understood. Carbon dioxide accumulation mechanisms are essential for algae, due to the low solubility of carbon dioxide in water.

Fig. 4.6: Carbon dioxide concentration mechanisms. Algae and plants have evolved different mechanisms to concentrate CO2 in the vicinity of RubisCO, and hence depress the side reaction of RubisCO with oxygen and allow higher rates of photosynthesis at a given external CO2 concentration. (A) Pumping of bicarbonate and release of CO2 via a carbonic anhydrase reaction located in the immediate vicinity of RubisCO in subcellular structures (carboxysomes in prokaryotic cyanobacteria, or pyrenoids in eukaryotic algae). (B) C4 Photosynthesis. CO2 is pumped via a cycle involving the synthesis of 4-carbon acids like malate in external (mesophyll) cells in the leaf and their movement to internal cells (bundle sheath) where they are decarboxylated and the CO2 is fixed via the Calvin-Benson cycle. (C) CAM Photosynthesis. In the dark, stomata are opened and CO2 is used for the synthesis of 4-carbon acids like malate which are accumulated in the vacuole. In the light, stomata are closed and malate is decarboxylated to release CO2 that is fixed via the Calvin-Benson cycle.
Higher plants use a different strategy to concentrate carbon dioxide. So-called C4 plants [24,25] initially incorporate carbon dioxide (actually bicarbonate) into 4-carbon organic acids like malate via a reaction that is catalyzed by phosphenolpyruvate carboxylase. This reaction occurs in cells in the outer part of the leaf. The malate diffuses into specially thickened cells in the middle of the leaf, where it is decarboxylated to release carbon dioxide, which is assimilated via RubisCO and the Calvin-Benson cycle. Because phosphenolpyruvate carboxylase has a very high affinity for bicarbonate and no side reaction with oxygen, photosynthesis can operate with much lower carbon dioxide concentrations in the internal air spaces in the leaf that are in direct contact with the stomata. This means that the stomata do not need to open so wide and that water loss is decreased. In Crassulacean Acid Metabolism (CAM) plants, phosphenolpyruvate carboxylase incorporates carbon dioxide into malate in the dark. Their cells have an extremely large central water-filled vacuole and can store concentrations of up to 1 molar malate. In the light, the malate is decarboxylated to release carbon dioxide, which is assimilated via RubisCO and the Calvin-Benson cycle. CAM allows water loss to be decreased because the stomata remain closed in the daytime and instead open at night when lower temperatures decrease evaporative water loss.
C4 and CAM plants have evolved multiple times in different taxa and families [22,25]. This probably occurred in response to intensive selective pressure during periods in the last 20 million years, when the atmospheric carbon dioxide concentration was often lower than today, sometimes falling to below 200 ppm [22]. The most recent “low carbon dioxide periods” were as recent as 10–12,000 years ago. These low carbon dioxide levels would have strongly restricted photosynthesis by C3 plants and provided strong selective pressure for plants with C4 or CAM photosynthesis.
So why do not all plants use one or the other of these mechanisms for carbon dioxide concentration? The answer is that carbon dioxide concentration mechanisms require energy. Under current atmospheric conditions C4 plants and CAM plants actually require more energy than C3 plants except at high temperatures, when the rate of photorespiration rises relative to the rate of photosynthesis [22]. At the present geological time, C3 plants can compete with C4 plants except under hot or dry conditions [12]. Most C4 plants are tropical and subtropical grasses that grow in seasonally dry zones, while most CAM plants are cacti that grow in semi-arid zones.
There is obviously intense interest in decreasing photorespiration because this could lead to an increase in photosynthesis and crop yield and also to a decrease in water use. For the past 30 years, the catalytic mechanism and structure of RubisCO have been studied with the hope of engineering a form with a higher specificity factor for carbon dioxide [26]. There has also been a longstanding interest in engineering C4 photosynthesis into conventional crops (most major crops are C3 plants, with the exception of maize, sugar cane and sorghum). This is a challenging task because it requires a fundamental change in leaf anatomy and the introduction of many different enzymes and transport proteins into specific cell types. However, the finding that individual components of the C4 pathway exist in C3 plants and the fact that C4 photosynthesis has evolved many times indicates that some basic genetic “switches” exist that underlie these complex morphological and biochemical changes. If the underlying “master genes” can be discovered, it may be possible to use them to engineer the multiple and complex changes that are needed to convert a C3 plant into a C4 plant [24]. Other approaches investigate if a more fundamental reengineering of metabolic pathways could lead to an increase in the efficiency of photosynthesis. Several projects are searching for a more efficient way to salvage 2-phosphoglycolate than the complex pathway that naturally evolved, probably in a piecemeal way as slowly changing atmospheric conditions led to its becoming an increasingly crucial issue for survival [21]. An even more radical approach is to use large scale pathway reconstruction and synthetic biology to try to create a completely novel carbon dioxide fixation pathway that does not require RubisCO [27].
4.4 The Use of Photosynthesis to Drive Plant Growth
Light energy-driven processes lead to the formation of sugars, amino acids, nucleotides, lipids and many other small metabolites. These resources serve to synthesize the macromolecules that build a cell—proteins, lipids and complex polysaccharides. Without going into detail, this involves thousands of biochemical reactions and transport steps, many of which lead to a loss of energy. Further, each reaction and transport step is catalyzed by a specific protein, which has to be synthesized, which again costs energy. For example, proteins are synthesized by molecular machines called ribosomes. In a rapidly growing microbial or plant cell, it is estimated that over half the cell’s resources are actually invested in ribosomes [28]. Cellular growth is a very capital-intensive process. Large scale investment of carbon, nitrogen, phosphate and energy is required to produce the machinery that itself is needed to convert the raw products (the immediate products of photosynthesis and the assimilation of mineral nutrients) into cellular structures. As a result, 30–60% of the assimilated carbon is respired to carbon dioxide in the remainder of the plant to provide energy for growth and for the maintenance of the cellular structures that are needed for growth processes [12].
4.4.1 Vegetative and Reproductive Growth: (Bio)Mass Production versus Quality Products
So far, the biochemical reactions converting the products of photosynthesis into the building procedure of a cell (proteins, lipids and complex polysaccharides) have been considered. However, this only captures one aspect of plant growth. A plant consists of many cells organized in different organs with different functions. This multi-functionality is important for the growth and survival of plants. However, it incurs further costs when plants are considered solely from the perspective of energy conversion. It also has important implications for what kinds of plants can be sensibly used as energy crops and how we must proceed to minimize competition between the production of bioenergy and food.
Figure 4.7 provides a simplified overview of the growth of a young plant. In mature leaves, light and
are absorbed and converted into carbohydrates like sucrose and starch. The roots absorb nutrients and water from the soil. These are moved in the transpiration stream (as discussed above) to the leaves and used, in combination with the products of photosynthesis, to make amino acids, nucleotides and many other small molecules. Sucrose, amino acids and nucleotides are then exported and used to support the growth of young leaves and roots. As a result, the plant acquires more leaves and a larger root system, which allows the plant to absorb even more light and
, and access more water and nutrients. This in turn will allow more photosynthesis at the level of the whole plant and even faster growth. This is basically like a bank account that pays compound interest. In contrast to most bank accounts, the interest rate is very high. A young plant under optimal conditions will often increase its weight by 20–30% per day. Thus, young plants typically show an exponential increase in their biomass, which doubles every 4–6 days (see, e.g., [29]).
As the plant becomes larger, this process may slow down because, for example, some of the leaves start to shade each other, or the roots start to exhaust the water or mineral nutrients in the soil, or an increasing proportion of the new growth has to be invested in structures that do not directly allow resource gain (e.g., thicker stems to prevent the plant from falling over). Nevertheless, plants continue to gain weight at rates that would thrill most dealers on Wall Street, if their stocks were to increase in value at a comparable rate.
Later, plants start to produce reproductive organs (Figure 4.7). This can involve the formation of flowers that, after fertilization, develop into seeds, berries or fruits. Some plants produce asexual reproductive organs like potato tubers, thickened storage roots like carrots or sugar beet, or rhizomes (e.g., many grasses).



Fig. 4.7: Schematic presentation of plant growth. In young plants, light energy drives the assimilation of
in leaves and the uptake and assimilation of inorganic nutrients by the roots, generating sugars, amino acids and other metabolites that are used to synthesize more leaves and roots. They, in turn, allow the acquisition of even more
, light, minerals and water, which in turn supports even more growth. In a young plant, growth is typically exponential in time, with an increase in biomass of 20% or more per day. Later in the life cycle, growth slows down or stops as resources are used to generate storage and reproductive structures. Investment in the formation of new leaves and roots is curtailed or stopped. This schematic representation reflects the life cycle of determinate annuals (plants that grow, flower and die within one year, i.e., most crop plants). Plants that grow for two or more years have more complex and flexible life cycles.
Reproductive organs are important for at least three reasons. First, they are vital for survival. Seeds and tubers allow annual species (i.e., plants that only live for one year) to survive seasonal periods of weather that would kill the mature plant, like the winter in northern or southern latitudes, or the dry season in climate zones with a hot dry summer. Second, seeds allow distribution of the species. This is important for plants as, unlike animals, they cannot crawl away or walk about. Seeds can be distributed by the wind (e.g., many grass seeds, dandelions, sycamore seeds), by water (e.g., coconuts) or by animals eating and excreting them. In the last case, the seed is usually surrounded by some attractive food for the animal such as berries and fruits while the seed coat is resistant to the animal’s digestive enzymes. The seed is then deposited at some site that the animal has wandered off to, together with a convenient dose of organic fertilizer. Third, sexual reproduction allows the mixing and recombination of chromosomes and thus reshuffles the genetic material and by doing so maintains its diversity.
Seeds contain large amounts of energy-rich storage compounds like starch, lipids and proteins. These nutritive and energy-rich resources are needed to support germination and the growth of the seedling. Starch is a polymer made from glucose and consists of a mix of linear α(1–4) and branched α(1–4)(1–6) glucan chains. It differs from glycogen, which is an important storage carbohydrate in many animals, and in humans as well, because it forms a crystalline structure which is insoluble and more resistant to microbial attacks than free sugar molecules.
Further resources are used to produce surrounding structures like the tough seed coat. In the special case of fruits and berries, resources are also used to make the surrounding nutritional sacrifice that tempts animals to swallow the seed.
An unavoidable result of flowering and seed formation is that plant growth —seen as an increase in weight—slows down or even stops. Indeed, biomass may even decrease. Existing leaves and stems may even enter a process called senescence, in which their protein, lipids and other components are degraded and exported to the growing seeds. Incoming light energy is no longer used to produce more leaves and roots. Instead, existing biomass is used to make high-quality, high-energy long term stores at a specific number of spatially defined sites. These stores are needed to support germination and the growth of the seedling in the following generation. An analogous process occurs during tuber formation.
In effect, instead of continuing to reinvest gains in a high-interest account, funds are cashed in and moved to the vault, incurring large bank charges [30]. The advantage of the plant’s strategy to stop growth and allocate resources to seed formation is clear: it is investing in the next generation. The large amounts of protein and energy-intensive storage compounds like starch and lipids will support the growth of the next generation long enough for the seedling to produce the necessary leaves and roots to fend for itself.
The cessation of growth during the formation of seeds and tubers is especially large in many crop plants. Our crop plants are typically annuals in which the vegetative organs die back as the seeds are being filled. They have been selected and bred over thousands of years to maximize the yield of seeds or tubers. One of the major ways in which this has been achieved was the increase of the so-called harvest index (the proportion of the biomass that is found in the part of the plant that is harvested to be used as food, i.e., the seeds or tubers). They have also often been bred to obtain synchronous flowering and seed growth because this makes it much easier to harvest fields with thousands of plants.
Mankind’s major staple foods are seeds from the Graminaceae—cereals like wheat, barley and rye—and from related plants like maize, rice and sorghum. Other important staple foods come from the protein-rich seeds of the Leguminoseae, like soybean, many different bean cultivars, peas and chick peas. Other important staple foodstuffs are based on starch-containing tubers like potato and cassava. Occasionally, fruits form an important staple, for example starch-rich bananas or plantains in some parts of sub-Saharan Africa. Oils also represent an important and energy-rich component in many diets. These are also largely obtained from seeds (e.g., rape seed oil, sun flower oil) or fruits (e.g., olive oil, palm oil). But why do we rely so much on seeds for food? The answer is three-fold.
First, seeds can be stored. They have evolved to survive not just one winter or dry season, but for many years. This is achieved due to the physical structure of their seed coat, but also to the fact that they are almost completely dried out. This will restrict growth of bacteria and fungi. In addition, many seeds actually contain defence compounds to ward off the unwanted attention of microbes and animals. Examples include derivatives of cyanide in cassava (also called manioc, a starch-rich root that is a major staple crop in sub-Saharan Africa) or strongly allergenic lectins in many legume seeds (beans, peas, etc.). Storability of the staple foodstuff was essential when mankind moved from being a hunter and gatherer to producing food from crops because growth of the latter depended on the season. One interesting cultural side-effect is the immense creativity that has gone into turning an object that is physically inedible (like a wheat grain) into something that is not only edible, but often delicious, and the cultural and culinary diversity that this has engendered.
Second, seeds and, to a lesser extent, tubers and some fruits, especially fruits from the tropics, provide a concentrated source of proteins, carbohydrates and/or lipids. In contrast, the remainder of the plant is mostly inedible. It may provide us with nice flavors, vitamins and roughage, but has very little nutritive or energetic value. This is partly due to another fundamental difference between the growth of plants and animals. Whereas animal cells are full of proteins or lipids, plants cells are like a large balloon: mature plant cells have a large central water-filled vacuole that typically occupies 90% of the cell, with a thin layer of protein around it where metabolism occurs (Figure 4.8).

Fig. 4.8: A schematic representation of a typical plant cell. The plant cell is surrounded by a thick cell wall that is shared with the neighboring cells. The cell wall consists mainly of cellulose and cell wall matrix components and provides rigidity and physical stability to the cell. The cell itself consists of a layer of cytoplasm and a large central vacuole. The cytoplasm consists mainly of proteins and nucleic acids. It is the site of most metabolic activity but occupies only about 10% of the cell volume. The cytoplasm includes smaller substructures like chloroplasts, mitochondria and the nucleus (not shown). The vacuole consists mainly of water, with dissolved ions and a few metabolites, and occupies about 90% of the cell volume. The cytoplasm is bounded by the plasmalemma, and the cytoplasm and the vacuole are separated by the tonoplast; both are thin membranes that are composed mainly of a phospholipid bilayer with embedded water-insoluble proteins, and provide a semipermeable layer between the cytoplasm and the external surroundings/cell wall, and the cytoplasm and the vacuole, respectively.
This means that, for a given amount of protein, a plant cell occupies a much larger volume and/or can generate a much larger area than an animal cell. This is obviously very useful for a sessile organism that cannot walk about but wants to maximize light and carbon dioxide absorption by generating a large leaf area. Similarly, the plant can generate a physically larger root system underground to access minerals and water in a large soil volume. Third, the bulk of a plant cannot be degraded by the human intestinal system. Plant cells are surrounded by the so-called plant cell wall (see Figure 4.8), which is indigestible to humans but nevertheless plays an important role by providing roughage to aid digestion. The plant cell wall has many functions in plants, including structural support and defence, which will be described in more detail in the next section.
Many current methods for generating bioenergy also use seeds or tubers as the feedstock to produce alcohol or biodiesel (see below). They do this for the same reason we use seeds and tubers for food; they are a high-quality and energy-intensive product that can be relatively easily processed and degraded. However, the use of carbohydrates and lipids in seeds and tubers as a starting point for bioenergy enters into direct competition with the production of staple foods for nutrition. It is a very wasteful way to produce energy because the production of seeds and tubers is inherently inefficient and because they only represent a small proportion of the total biomass of the plant.
4.5 Vegetative Biomass: What to Do with the Cell Walls?
Why is the rest of the plant not used for bioenergy production? As already mentioned, a typical mature plant cell contains a lot of water and a relatively small amount of proteins, lipids or carbohydrates. The vast bulk of dry vegetative plant biomass derives from the cell walls that surround the cells and are much more difficult to degrade than carbohydrates or lipids. The cell wall provides structure to the plant cell, which otherwise would be a jelly-like bag of water. It also provides structure at the level of the organ and the whole plant. Plants do not have bones, instead, their physical stability results from the walls of all of their individual cells. In small plants, each cell is firm because the ions and small metabolites within the cell act via osmosis to draw water into the cell. This generates a pressure (called turgor) that is exerted against the cell wall. If the cell wall is experimentally removed, the cell will actually explode, unless it is surrounded by a 4–500 mmolar solute. The firmness of a salad leaf is due to all of the cells pushing against their cell walls, and the cell walls of each cell pushing against the cell walls of its neighbors. Plants also possess specialized cells that make especially thick cell walls and provide physical support, which is independent of the interplay with water. These cells play a role in allowing the plant to move water from the roots up into the leaves in a transpiration stream. This is done via a long file of dead cells called xylem vessels, whose thick walls prevent them from collapsing inward as water is sucked up from the roots. In larger plants, these cells become far more numerous and provide large supportive tissues. This is seen most dramatically as wood in the stems of trees.
The plant cell wall is a complex macromolecular structure. As might be expected from its role in providing rigidity and defence, it is extremely difficult to degrade [31-34]. The main component of the cell wall in non-woody plants is cellulose, which is estimated to represent about 30% of the dry weight of a plant. Cellulose, like glycogen or starch (the major carbohydrate stores in animals and plants, as mentioned above), is a polymer made of glucose. However, it differs in that the glucose residues are linked via a β(1–4)-glycosidic link rather than an α(1–4)-glycosidic link. This apparently small change in the stereoisometry (spatial structure) of the chemical bond that links the adjacent glucose molecules has a major impact on the spatial organization of the polymer. In glycogen and starch the glucose polymers form a loose helix. In cellulose the β(1–4)-glycosidic link is reinforced by an H-bond between the OH-group on the C3 atom of one glucose residue and the oxygen of the O-glycosidic bond. This prevents rotation around the O-glycosidic bond. As a result, the lowest energy conformation for the polymer is that of a linear ribbon-like structure. These can then stack on top of each other, with H-bonds between the adjacent chains leading to the formation of a microfibril. A microfibril is estimated to contain about 36 individual macromolecules, each 500–1400 glucose residues long. These long and inelastic microfibrils wrap around cells in spatially oriented and overlapping layers. They are able to withstand pressures of the same order as those in a car tire [33]. The cellulose microfibrils are held together by several other classes of macromolecules, including hemicelluloses, pectins and glycoproteins. These macromolecules are more flexible, with segments that loop between the cellulose microfibrils, and others that bind onto sites along the cellulose microfibrils [33,35,36]. A loose analogy would be to imagine the cell wall as reinforced concrete, with the cellulose being the steel rods and the hemicelluloses, pectins and glycoproteins the concrete that holds them in place.
In wood, the cell wall is further strengthened by the deposition of lignin. Lignin is a highly complex macromolecule formed from various types of aromatic phenylpropanoid molecules. The variety of subunits and the fact that they do not necessarily polymerize in a fixed order makes lignin rather unusual due to its heterogeneity and lack of a defined primary structure. It is even more difficult to degrade than cellulose. Lignin is one of the most abundant organic polymers on earth, exceeded only by cellulose, employing 30% of non-fossil organic carbon and constituting approximately a quarter to a third of the dry mass of wood [37]. Together, lignin and cellulose make up well over half of the plant matter on earth. Jointly, they are often termed lignocellulose.
As already mentioned, the digestive tract of humans is not able to degrade cellulose. Vegetables like lettuce and cabbage and fruits like apples and oranges are important sources of vitamins, minerals and roughage, but contain negligible amounts of energy. However, there are obviously herbivores—animals that eat only plants. Many of these mainly feed on leaves (in particular grass) and are able to digest cellulose. Examples are ruminants like cows, sheep, deer, camels and antelopes whose digestion of cellulose in their rumen depends on a dense population of bacteria, protozoa, and fungi. These produce an enzyme called cellulase that is able to degrade cellulose, and a further cocktail of enzymes that converts cellulose into the disaccharide cellobiose. The latter is fermented in the largely anaerobic rumen to acetate, lactate, propionate, butyrate, carbon dioxide and methane. Other examples of herbivores are the so-called end-gut fermenters like rabbits and horses. They ferment cellulose in an extended large intestine (caeceum) and if necessary re-ingest soft pellets (caecal) that contain well fermented microbial products. The relation between such herbivores and their microbial gut inhabitants is a good example of a symbiosis where organisms gain a mutual benefit from a partnership. There is an analogous symbiosis with other microbes in insects that are able to digest the cellulose and lignin in wood; with termites as a well-known example.
This raises the question whether these specialized microbes can be used to convert plant cell wall material and wood into products, which can then be fermented by yeasts to alcohol or other products that can be used as energy. Such processing could, in principle, allow a complete or near-complete conversion of vegetative plant biomass into usable compounds. There is intensive research worldwide into such processes, as described in other chapters of this book. In parallel with research in white biotechnology and chemical engineering, there is also increasing research into the functional properties of the plant cell wall to identify features which might be changed in order to render it less intractable to chemical and enzymatic digestion [32,38].
There is also interest in developing other ways to use lignocellulose, as an alternative to its conversion to alcohol or other burnable liquid fuels. At this time, biogasification is the most energy efficient way to convert plant biomass into burnable compounds. Biogasification also depends on specialized bacteria and results especially in the formation of methane. Methane is, however, itself a very effective greenhouse gas. This means that widespread use of this technology will depend on engineering and processing methods that near-to-completely prevent its release into the environment. Another approach, which is feasible with dry plant matter, is to burn it. A further interesting process is hydrothermal carbonization to process wet biomass [39]. Low value biomass like garden waste and leaves, crop residue, liquid manure, horse droppings and residues from many industrial food production processes can be converted into products like peat or coal within a few hours. Because the process is exothermic (i.e., producing rather than consuming heat) it requires only a small initial energy input, after which it produces usable heat. As will emerge later in this chapter, we will probably need to use a wide range of different crops as feedstocks for bioenergy. This will place a premium on having a range of methods to process the plant material, or methods that are robust to changes in the composition of the plant matter.
4.6 Response of Photosynthesis and Plant Growth
to Rising Atmospheric Carbon Dioxide
One of the drivers of climate changes is the rising atmospheric carbon dioxide concentration. As already mentioned, the rate of photosynthesis is increased by rising atmospheric carbon dioxide, especially in C3 plants. This opens the hope that in the future, crop yield will increase as a (beneficial) consequence of rising atmospheric carbon dioxide concentrations. Further, we might hope that rising rates of photosynthesis in natural ecosystems could lead to faster growth and sequestration of more carbon in standing plant matter or as dead plant matter in the soil. These hopes were supported by early studies under artificial laboratory or greenhouse conditions, which on average indicated a potential yield gain of over 30% as the atmospheric
concentration is increased from present-day levels up to 550 ppm
, which is the projected level in 2050. To put this into perspective, projections of the increase in crop yield that will be needed to match the increasing demand for food lie between 40–80%. Does this mean that the increasing carbon dioxide concentration will automatically solve many problems?
In the last 15 years, this possibility has been analyzed more rigorously in a large number of large scale Free Air Carbon dioxide Enhancement (FACE) studies with many crops, including tree plantations, at different locations around the world. These studies have confirmed that there is a stimulation of photosynthesis of ca. 30%, which is in line with predictions made from photosynthesis models. As plant growth is quasi-exponential, this stimulation of photosynthesis should translate into an even larger increase in yield. However, the average yield gain shown in FACE experiments was about 14% [40-42]. Further, in a small number of studies in which elevated carbon dioxide was combined with other predicted changes due to climate change (e.g., increased temperature) or anthropogenic activity (e.g., ozone), the increase in yield was abolished.
The rather small response of yield to elevated carbon dioxide implies that there are major restrictions on growth downstream of photosynthesis. These will include constraints by external factors, like nutrient availability or water, which will become exhausted more rapidly if a plant grows more rapidly. However, there are probably also internal developmental or genetic constraints on the extent to which the growth of a given plant can be increased.
Up to now FACE studies have been performed with one or a very small number of cultivars. It is therefore unclear if the small yield is always due to a weak growth response to carbon dioxide, or also to the specific lines that were used while other lines might respond more. In particular, it is unclear if there is any genetic variation in the breeding populations which would allow the gain in photosynthesis to be transformed into a gain in yield. This raises a dilemma. For many traits, it is possible to carry out breeding trials in different locations to look for lines that will perform better in a given condition. For example, large populations of plants can be screened to identify lines that perform well at low temperatures or with less water by having a set of field sites that differ in temperature or rainfall. At present, there are no FACE sites large enough to perform this kind of large scale screening of our breeding resources for responses to future carbon dioxide levels. Planning and developing such sites is an important challenge for the future [40-42].
4.7 Integrated Model of Energy Use Efficiency
during Photosynthesis and Growth
The preceeding sections discussed the processes of photosynthesis and growth, and highlighted that the energy conversion efficiency is low, partly due to the inherent need for a loss of energy in any process and partly because of a series of design features which lead to an additional loss—or wastage—of energy. Quantitative analyses by Zhu et al [12] and Blankenship et al. [11] predict that the maximum efficiency of photosynthesis (conversion of energy in the incident light into carbohydrates as the products of photosynthesis) under current atmospheric conditions is of the order of 5 and 8% for C3 and C4 plants, respectively. The predicted maximum efficiency for the conversion of incident light energy into vegetative biomass is below 2 and 2.5% for C3 and C4 plants, respectively. The actual efficiency will be much lower, because the light is sometimes saturating, temperatures may not be optimal for growth and growth may be limited by the availability of nutrients or water. It will become even lower when plants enter the phase of reproductive growth.
Figure 4.9 recapitulates and simplifies the calculations of Zhu et al. [12] and Blankenship et al. [11]. This simplified calculation starts with the value of 120,000 TW of energy, which is the total annual solar energy that is intercepted by the earth. It first considers how much of this irradiation is intercepted by plants and then uses the information about the energy conversion efficiency of photosynthesis and growth to estimate how much of this energy is conserved in plant biomass. Complex and partly unresolved questions about the precise parameterization of the individual process, especially energy conversion in the dark reactions and in plant growth, are simplified for the purpose of illustration by setting the energy loss at most of the steps at 50%. They will anyway vary from plant to plant, region to region and day to day. The efficiency cannot be more than two times higher than 0.5, and is also probably not more than two times below it. More precise values are retained for the energy conversion efficiency of light absorption and the light reactions because these processes can be more precisely parameterized with experimental or theoretical values (see [11,12]).

Fig. 4.9: Schematic representation of the conversion of solar energy by the biosphere. This scheme recapitulates and simplifies the calculations of Zhu et al. [12] and Blankenship et al. [11]. Complex, and in part unresolved, questions about the precise parameterization of the individual process like the energy conversion efficiency of the dark reactions and of plant growth are simplified for the purpose of illustration by setting the energy loss as 50%.
This simple exercise predicts that primary biomass production per year will have an energy content of the order of 100 TW. This is only 0.08% of the energy in the total incoming radiation that arrives at the surface of the earth. The energy content of the resulting biomass is about 0.8% of the energy in the sunlight that is intercepted by plants. This value is about two-fold lower than the maximum values estimated by Zhu et al. [12] because my schematic model already assumes that light is sometimes saturating for photosynthesis.
The calculation in Figure 4.9 puts my earlier optimistic comments about the global availability of solar energy into a stark perspective. Comparison of the estimated primary biomass production (~100 TW p.a.) with the total current (15 TW p.a.) and projected (30 TW p.a.) global energy needs of mankind shows that there are limits to how much of the total energy requirement can be met by bioenergy.
As pointed out by Blankenship et al. [11], the energy conversion efficiency of photosynthesis and plant growth is much lower than the energy conversion efficiency of photovoltaics (in good cases larger than 20%). It is instructive to ask why plants are apparently so inefficient. One reason has already been mentioned, both in the context of the light reactions and of RubisCO. Photosynthesis was not primarily designed to maximize energy conversion efficiency. It was developed in the context of, and had to be compatible with, a pre-existing network of reactions and proteins. Although selection can lead to the evolution of increasing effective living systems, it cannot start again from scratch. However, there are also other and deeper reasons why plants cannot be as efficient in energy conversion per se as a man-made device. Man-made devices are assembled by men (or by machines made by men). The materials needed to construct them are collected and pre-processed by men. The devices are repaired by men (or machines made by men). If the devices are damaged by external events or break down due to wear and tear they are repaired by men. If they break down irretrievably they can be replaced by new machines, which are made by men. Further, machines do not have to improve themselves. This is done by men, who plan and produce the next model, or a new sort of machine that does things in a better way. In contrast, living organisms have to be self-assembling and self-repairing. They have to reproduce themselves and, over time, must evolve to meet the challenges posed by changes in their biological, chemical and physical surroundings. These self-sustaining activities come with an energy cost and place constraints on the design of living organisms.
4.8 What is Needed for Efficient Energy Crops?
The preceding sections lead to the following requirements for an efficient energy crop:
1It should not compete with food crops.
2As far as possible the entire biomass of the plant should be used as a feedstock for bioenergy, which will require the development of methods to efficiently utilize lignocellulose.
3It should be grown using methods that minimize energy-intensive chemical inputs.
4It should as far as possible be grown on land that is marginal for food crops and/or the feedstocks for energy production should be derived from parts of the plant that are not eaten.
4.9 Current Energy Crops and Their Shortcomings
Most current methods for generating bioenergy use seeds or tubers as feedstock. These are often referred to as “first generation” energy crops. Starch or oil-rich seeds and tubers are used as the feedstock (starting point) for bioenergy production because they provide a concentrated source of high-energy precursors that can be converted to fuel by a combination of physical, chemical and microbial (fermentation) approaches. One major source of biofuels is the use of maize, cereal seeds and sugar beet roots to produce bioethanol. The most widely used procedure to convert sugar- and starch-rich plant matter into ethanol takes advantage of the fact that many yeasts ferment carbohydrates to alcohol. Fermentation is a strategy to cope with anaerobic conditions and allows yeast to convert a small part of the energy in carbohydrates into ATP while producing alcohol as a waste product. Another major source of biofuels is to use oil-rich seeds or fruits like rapeseeds and palm oil to to produce biodiesel. Biodiesel is typically made by chemically reacting lipids, for example vegetable oil and animal fat (tallow), with alcohol to generate long-chain alkyl (methyl, propyl or ethyl) esters.
The methods used to produce the majority of these so-called “first generation” biofuels have several major drawbacks. First, the use of seeds and tubers involves direct competition with staple food production. Second, the methods used to produce the feedstock are inherently inefficient. The starting point is a high-quality product whose formation is inherently inefficient because the plant decreases or even stops growth during the formation of seeds and tubers. Further, growth of the crops often occurs in an intensive manner, requiring the use of large amounts of fertilizer. Depending on the crop, the geographical location and the inputs and outputs used in the analysis, the energy balance can be positive or negative [43].
The most successful current source of bioenergy is sugar cane. The 26 billion liters of ethanol produced in Brazil in 2010 represent about 30% of the total ethanol used as fuel in the world at present [44]. Sugar cane is a successful energy crop for several reasons. First, it is a C4 plant, with inherently high rates of photosynthesis (see above). Second, it is a tropical and subtropical crop that can be grown all year round, maximizing absorption of incident light. Third, sugar cane is unusual in that it stores large amounts of sugars in its stem during vegetative growth. While originally selected and used to produce sugar as a foodstuff, sugar cane also provides an excellent feedstock for the production of ethanol.
However, a large part of sugar cane biomass is not fermented but remains behind as a waste product (bagasse) which consists to about 50% of cellulose, 20% of lignin and the rest mainly made up of other cell wall components. Bagasse is also a waste product of sugar fermentation; for each 10 tons of sugar cane crushed, a sugar factory produces nearly 3 tons of wet bagasse. It often accumulates in large mounds near the sugar mill. There have been large efforts to use bagasse as a starting point for making other products or energy, and these illustrate some general issues in the use of vegetative plant biomass as a feedstock for energy production. First, its composition can be highly variable. Second, its high moisture content (40 to 50%) makes it costly to move and difficult to use as a fuel. Clearly, solving these problems would further improve the energy, economic and ecological balance of sugar cane as a bioenergy crop.
In 2009, the 4.6 Mha of land cultivated for sugar cane in Brazil allowed the production of about 27 gigaliters of alcohol, plus about another 2 gigawatts of electricity via the combustion of bagasse [45]. This is already equivalent to about 40% of the petrol used in Brazil. It is planned to expand sugar cane cultivation to a maximum of 63.5 Mha, mainly by increasing cattle stocking density on a much larger area of about 237 Mha that has already been cleared and is currently being used for cattle ranching (Decree No 6.961, September 17, 2009, Diário Oficial União de 18.9.10, Brazil). This would allow expansion of the area used for this bioenergy crop without further clearing of natural ecosystems. Assuming that advances in the processing of plant biomass also allow the full use of bagasse to produce a liquid fuel, Somerville et al. [38] estimated that Brazil could produce up to approximately 800 gigaliters of ethanol per year, equivalent to approximately 14% of the current world transportation fuel demand (4900 gigaliters) in 2006.
4.9.1 Perspectives for Improving Current Crops to Optimize
Bioenergy Production
Two complementary routes are available to improve energy crops. One is to use changes in plant breeding and agronomic practice to improve the energy and ecological balance of existing crops. Another is to develop new dedicated energy crops (see next section).
I will discuss maize as an example of how an existing crop might be made more efficient for energy production. Maize has the advantage that it is already a high-yielding crop which produces a large standing biomass per unit area. In addition, it is one of the crop plants that lends itself relatively easily to crop breeding because it contains a large amount of genetic diversity in its breeding material. In terms of global grain or seed production, maize is the largest crop, producing about 820 million metric tons of grain for food and animal feed purposes. Maize seeds contain a high starch and (in the case of sweet corn) sugar content. There is also a similar amount of stems and stripped cobs (stover) potentially available for fuel production.
One option to increase biomass would be to delay or suppress flowering in order to maximize the duration of vegetative growth and biomass formation. Flowering is a crucial transition in the life of a plant. The timing of flowering is regulated by many inputs including the day length (as an indicator of the time of year), temperature, nutrient status and endogenous signals related to the age of the plant. Maize flowers when the length of day decreases below a critical threshold. This can be seen as a strategy to initiate flowering as autumn approaches. Day length is a more accurate predictor of the time of year than temperature, which fluctuates from day to day and year to year. Within a species, there can be a large genetic diversity in the response of flowering to the length of day. This allows adaptation to the location where the plants are growing. On a given day of the year, the day length depends on the latitude; for example, in the summer months, plants growing at latitudes further away from the equator will experience longer days than plants that grow closer to the equator. Plants at high latitudes are, however, also likely to be subjected to an earlier autumn and winter. They therefore need to flower earlier even though they are experiencing longer days. The wild progenitors of maize grew at different latitudes and altitudes, and different progenitors carried different genes and alleles (variants of genes) that are adapted to these different locations. Enough of this genetic diversity was retained during the domestication of maize to allow the selection of cultivars that are adapted to different latitudes. When plant breeders develop maize cultivars for a particular geographical location, they use this genetic diversity to select an appropriate breeding response to maximize vegetative growth but also ensure that cob maturation is completed before temperatures fall in the autumn. This requires cultivars that are bred for higher latitudes to flower at longer day lengths than cultivars that are grown nearer the equator. To breed “energy maize” designed for temperate regions like Northern Europe, the selection criteria can be changed and plants selected that do not flower until the day length is much shorter. These plants will not develop cobs because they do not flower until early autumn. This can be achieved by either crossing cultivars adapted to Northern Europe with cultivars selected for lower latitudes (e.g., Italy) or by crossing the Northern European cultivars back into “land” races or wild progenitors from the highlands of Middle America or the equatorial Andes.
A complication is that it is seldom possible to breed for just one trait. At best, other important traits have to be maintained and often several traits need to be improved simultaneously. The growth of maize in higher latitudes is restricted by temperature, especially the low temperatures in late spring which can slow down and even seriously damage young maize seedlings. This is the main reason why maize is not sown until that time. As a consequence, it does not form a closed canopy and absorb all the incident sunlight until early summer—much later compared to cereals like wheat and barley. Over the last decades, considerable progress has been made to improve this low tolerance. Such traits must obviously be retained through the crosses with maize cultivars for the use in warmer climate zones and further improved if possible.
Notwithstanding the possibilities of obtaining energy more effectively from the existing crop plants, the basic problem remains that these will continue to compete for land with food crops. As pointed out by Somerville et al. [38], even the extension of strategies for the use of crop plants like maize to include the harvesting and use of waste products like corn stover (stem and leaf material) may not have a major impact on energy efficiency. This is partly due to the additional financial costs incurred by harvesting and transporting the stover. In addition, the removal of stover may increase soil erosion and decrease the recycling of carbon and nutrients to the soil. For this reason, there is a need for a further and even more radical approach to develop a series of new crops that are specifically designed for the production of energy and chosen for their abilities to grow on land unsuited for food production and to thrive under agronomic methods that minimize energy inputs and negative environmental impact.
4.9.2 Novel Dedicated Energy Crops
Of the over 4000 plant species that humans have used in the past millennia as food, feed or source of other products, almost all were abandoned [46]. Current arable agriculture is based on a small number of species only. The diversification of the species used for crops is a major future challenge for modern plant science, not only with energy crops but also more widely [47].
The domestication of cereals was the central achievement of the Neolithic revolution 12,000 years ago in southeast Turkey [48]. This and the parallel domestication of maize and members of the Solanaceae (nightshade family) in pre-Columbian America, and rice and soybean in East Asia, shaped the food production on the planet [49]. Recent research has shown that domestication included the choice of alleles of specific genes that support an appropriate timing of seed germination, reduce the influence of the length of day on the timing of fruit ripening, improve seed size, keep the seeds in the ears and aid hand threshing of the ears [50]. Many of these traits are irrelevant, or even counterproductive, for energy crops. It will be an exciting challenge to see whether an increased understanding of plant function and genetics will allow new energy crops to be developed in the next decades, emulating advances that have required thousands of years for today’s crop plants.
Perennial grasses, in particular C4 grasses such as sugar cane, energy cane, elephant grass, switchgrass, and Miscanthus, are one likely source of future energy plants [38]. Three features of these grasses make them attractive candidates for energy crops. First, they have intrinsically higher light, water, and nitrogen use efficiency than C3 species [51]. Indeed, the highest annual dry-matter production reported for any vegetation to date is for such C4 grasses, including 88 MT/ha per year (MT = metric tons of dry weight) for Napier grass (Pennisetum purpureum) in El Salvador and 100 MT/ha per year for natural stands of Echinochloa polystachya on the Amazon floodplain [52]. These are rates of biomass production reaching the maximum possible rate of growth, based on incident sunlight and models for energy conversion efficiency developed by Zhu et al. [12]. Second, these plants grow in the warm and/or wet part of the year and develop a large standing above-ground biomass, which then senesces. The majority of the minerals move back from the shoot into storage roots. The above ground biomass can be harvested each year in the dry season or winter for conversion to bioenergy. The following year, shoots re-grow from the roots, using nutrients that are recycled form the previous year. This decreases the need for fertilization and, because ploughing is not necessary, decreases soil erosion. Further, the roots may add carbon to the soil. Third, these types of plants grow in many different types of ecosystems, from river swamps to semi-arid prairies and steppes. This is important, because it may make it possible to develop different crops for different marginal land that is unsuited for the use of food production.
For temperate zones, there is much interest in the perennial C4 grass Miscanthus x giganteus. In trials in England, this C4 grass generated a peak biomass of 30 MT/ha per year and a harvestable annual biomass of 20 MT/ha, the highest results recorded for a cool temperate climate. In side-by-side trials in central Illinois, unfertilized Miscanthus x giganteus produced 60% more biomass than a well-fertilized highly productive maize crop, and across the state, winter-harvestable yields averaged 30 MT/ha per year ([53,54], see also http://www.biofuelstp.eu/crops.html). For warm dry zones, switch grass from the North American prairies is attracting considerable attention. Arundo donax (giant reedgrass or Spanish cane) is considered a promising species for biomass production in Europe.
More than 600 Mha of land worldwide has fallen out of agricultural production, mostly in the last 100 years [38]. Some of this area appears suitable for the production of such perennial grasses or other types of energy crops. However, more research is necessary to categorize this abandoned land with respect to its potential for the various types of energy crops. Ironically, in some cases their use may be limited by poor infrastructure for transport of the feedstock or the fuel.
Trees provide a complementary source of biofuel feedstock. The potential of wood is illustrated by the fact that the wood biomass harvested each year in the Northern Hemisphere has an energy content that is equivalent to the annual liquid fuel consumption in the United States [55]. Of course, large natural forest and jungle areas should not be cleared because they form vital ecological habitats, are important for the climate and represent important stores of sequestered carbon (see above). However, there are strategies for sustainable felling of extended forest areas for lumber and paper production, which could be extended to providing feedstock for the production of energy. A major problem may be the lack of transportation and infrastructure in remote forested areas.
There are also considerable land areas that were deforested in the past and used for agriculture but were later abandoned and are now returning to their former forest state. Field and his co-workers estimate that, globally, 89 to 107 Mha of land that were once agriculturally used are now either forests or urban areas [56]. Of these, large tracts of forest land are now mature ecosystems. These are of high intrinsic value for their biological diversity for recreation and also because they are already sequestering carbon. However, more recently abandoned lands have lower ecosystem service values and could be used for the plantation of biomass crops [38].
The best practice to maximize woody biomass production per hectare appears to be coppice harvesting. This practice goes back to ancient times. It involves cutting fast-growing tree species like willow or poplar at near ground level after the end of the growing season every three to five years, depending on the species and the growing conditions. The plants rapidly regenerate shoots from the rootstock without any intervention. This approach minimizes losses of mineral nutrients, soil erosion, and organic carbon emissions. There are many similarities between this approach and the use of perennial grasses (see above). Typically, coppiced woodland is harvested in sections on a rotation with the result that a crop is available each year somewhere in the woodland. When managed this way, coppicing has the added advantage that it generates a variety of habitats, as the woodland always has a range of different-aged coppice growing in it, which is beneficial for biodiversity.
Almost 20% of the global terrestrial surface is classified as semi-arid with 200 to 800 mm of rainfall per year and an average growing season temperature larger than 21°C [43]. This land is little-used for food production, except when artificial irrigation is available. Indeed, much of the land that has fallen out of agricultural production worldwide is semi-arid [56]. There is interest in finding species that could grow under such conditions, even if their growth is relatively slow [38]. One example is the various Agave species, which grow well under semi-arid conditions. Like many other plants that are adapted to such conditions, they use CAM photosynthesis (see above). Textbook knowledge states that CAM plants grow slowly. However, some Agave species have been reported to exhibit surprisingly high harvested biomass yields of 7–10 MT/ha per year on semi-arid land when harvested at 5 to 6-year cycles [57,58].
All of the above scenarios are based on the use of plant cell walls as an energy source. These are basically complex carbohydrates and lignin. On a weight basis, however, lipids provide almost double the energy that is provided by carbohydrates. Whereas vegetative plant matter sometimes accumulates large amounts of carbohydrates (e.g., sugar cane, see above) and mutants accumulating large amounts of starch can easily be obtained [16], plants do not accumulate lipids in their vegetative tissues. Lipids are normally stored in seeds. There is considerable interest in understanding why lipids are accumulated only in this manner and also in using this knowledge to develop plants that store large amounts of lipids in mature vegetative tissues (e.g., see http://www.bch.msu.edu/faculty/benning/fulltextBenning.html). Another alternative is to identify plants that accumulate large amounts of lipid in their seeds or fruits, but grow on marginal land, like Camelina sativa, a plant that is cold-tolerant and has an oil yield of 35–38%, or Jatropha curcas, which is a tropical plant with a high drought tolerance and seeds with high oil content (approximately 40%).
There is also large interest in the potential of algae as a bioenergy feedstock. Provided they receive enough sunlight and minerals, algae can have much faster growth rates than terrestrial crops, and some species of algae can even produce up to 60% of their dry weight in the form of oil. Many of the challenges in the use of algae lie in the design and operation of efficient fermenter systems. A key advance would be to breed or engineer algae that secrete oil into the surrounding medium because this would make it less cost- and energy-intensive to harvest the oil.
Given that it will be an imperative to grow energy crops on marginal land with minimal input, the choice of energy crops will need to be oriented toward the climatic and edaphic conditions in each region. As pointed out by Somerville et al. [38], in many cases water availability may be a key factor, with the less water efficient C3 species like poplar and willow being grown in areas with sufficient rainfall, more water-efficient crops like sugar cane, switch grass and Miscanthus being grown where rainfall is not in excess, and specialized plants like Agave being grown in more arid regions. Likewise, in higher latitudes, temperature tolerance will be an important determinant.
Quoting Somerville et al. [38]:
Importantly, by focusing on the use of dedicated energy crops—rather than on repurposing food and feed crops—it should be possible to overcome many of the problematic constraints associated with our narrow dependence on a relatively small number of food crops and to develop agroecosystems for fuel production that are compatible with contemporary environmental goals.
This scenario points to the need for a diverse portfolio of species and varieties of these species. It also entails that there will be a wide range of plant feedstocks. Variation in the feedstocks from field-grown plants is probably also unavoidable due to year-to-year changes in the weather and change that may occur during storage for different durations. This, of course, will pose a challenge to the processes further downstream that are required to use these feedstocks.
Acknowledgments
I am grateful to the Max Planck Society for supporting my research, to the members of my research group past and present and to many colleagues with whom I have interchanged ideas about photosynthesis and plant productivity in the last years, especially Julian Hibberd, Steven Long, Thomas Sharkey and Andeas Weber.
[1] N.E. Borlaug. How to Feed the 21st Century? The Answer is Science and Technology.. In: Genetics and Exploration of Heterosis in Crops Ed. by G.J. Coors, S. Pandy. Madison, WI: ASA, CSSA, SSSA, 1999. .
[2] B. Halweil. Farmland Quality Deteriorating. In: Vital Signs: The Trends that are Shaping our Future New York: W. W. NortonCompany, 2002. 102-103.
[3] D.F. Calderini, G.A. Slafer. Changes in Yield and Yield Stability in Wheat During the 20th Century Field Crops Research (1998):.
[4] K.G. Cassman, A. Dobermann, A. D., Walters A.. Meeting Cereal Demand While Protecting Natural Resources and Improving Environmental Quality Annual Review of Environment and Resources 28: 15-58 (2003): 15-58.
[5] A.J. Cavalieri, O.S. Smith. Grain Filling and Field Drying of a Set of Maize Hybrids Released from 1930 to 1982 Crop Science 25: 856-860 (1985): 856-860.
[6] D.B. Egli. Comparison of Corn and Soybean Yields in the United States: Historical Trends and Future Prospects Agronomy Journal 100: 79-88 (2008): 79-88.
[7] P.L. Pingali, M. Hossain, M. H.. Asian Rice Bowls: The Returning Crisis. Wallingford, UK: CAB International, 1997
[8] D.N. Duvick, K.G. Cassman. Post-Green Revolution Trends in Yield Potential of Temperate Maize in the North-Central United States Crop Science (1999):.
[9] J.M. Beman, K.R. Arrigo, K. A.. Agricultural Runoff Fuels Large Phytoplankton Blooms in Vulnerable Areas of the Ocean Nature 434: 211-214 (2005): 211-214.
[10] J. Giles. Nitrogen Study Fertilizes Fears of Pollution Nature 433: 791 (2005): 791.
[11] R.E. Blankenship, M. David, M. D., Tiede M., T. M., D.M. Tiede, D. T., Barber D.M., B. D., J. James Barber, J.J. B., Brudvig J. James, B. J.J., G.W. Brudvig, G. B., Fleming G.W., F. G., G. Fleming, G. F.. Comparing Photosynthetic and Photovoltaic Efficiencies and Recognizing the Potential for Improvement Science 332: 805-809 (2011): 805-809.
[12] X.-G. Zhu, S.P. Long, S. L.. What is the maximum efficiency with which photosynthesis can convert solar energy into Biomass? Current Opinion in Biotechnology 19: 153-159 (2008): 153-159.
[13] H. Michel. Die natürliche Photosynthese: Ihre Effizienz und die Konsequenzen. In: Die Zukunft der Energie: Die Antwort der Wissenschaft Ed. by P. Gruss, F. Schüth., 2008. .
[14] J.A. Bassham. Mapping the Carbon Reduction Cycle: A Personal Retrospective Photosynthesis Research 76: 37-52 (2003): 37-52.
[15] A.A. Benson. Following the Path of Carbon in Photosynthesis: A Personal Story Photosynthesis Research 73: 31-49 (2002): 31-49.
[16] M. Stitt, B. Usadel, B. U.. Primary Photosynthetic Metabolism – More than the Icing on the Cake Plant Journal 61: 1067-1091 (2010): 1067-1091.
[17] C.B. Field, M.J. Behrenfeld, M. B., Randerson M.J.. Primary Production of the Biosphere: Integrating Terrestrial and Oceanic Components Science 281: 237-240 (1998): 237-240.
[18] E.H. Murchie, M. Pinto, M. P.. Agriculture and the New Challenges for Photosynthesis Research New Phytologist (2008):.
[19] G.H. Lorimer, T.J. Andrews. Plant Photorespiration – Inevitable Consequence of Existence of Atmospheric Oxygen Nature 243: 359-360 (1973): 359-360.
[20] C.R. Somerville. An Early Arabidopsis Demonstration. Resolving a Few Issues Concerning Photorespiration Plant Physiology 125: 20-24 (2001): 20-24.
[21] C. Peterhansel, I. Horst, I. H., Niessen I., N. I., M. Niessen, M. N.. Photorespiration. The Arabidopsis Book., 2010
[22] R. Sage. The Evolution of C4 Photosynthesis New Phytology 161: 341-370 (2004): 341-370.
[23] G.G. Tcherkez, G.D. Farquhar, G. F.. Despite Slow Catalysis and Confused Substrate Specificity, all Ribulose Bisphosphate Carboxylases may be Nearly Perfectly Optimized Proceedings of the National Academy of Sciences of the United States of America 103: 7246-7251 (2006): 7246-7251.
[24] N.J. Brown, C.A. Newell, C. N., Stanley C.A., S. C., S. Stanley, S. S.. Independent and Parallel Recruitment of Pre-Existing Mechanisms Underlying C4 Photosynthesis Science 331: 1436-1439 (2011): 1436-1439.
[25] J.M. Hibberd, E.E. Sheehy, E. S.. Using C4 Photosynthesis to Increase the Yield of Rice – Rationale and Feasibility Current Opinion in Plant Biology (2008):.
[26] S.M. Whitney, R.L. Houtz, R. H.. Advancing our Understanding and Capacity to Engineer Nature's CO Plant Physiology 155: 27-35 (2011): 27-35.
[27] A. Bar-Even, E. Noor, E. N., Lewis E.. Design and Analysis of Synthetic Carbon Fixation Pathways Proceedings of the National Academy of Sciences of the United States of America (2010):.
[28] J.R. Warner. The Economics of Ribosome Biosynthesis in Yeast Trends in Biochemical Sciences 24: 437-440 (1999): 437-440.
[29] Y. Gibon, E.-T. Pyl, ET. P., Sulpice E.-T., S. ET., R. Sulpice, R. S.. Adjustment of Growth, Starch Turnover, Protein Content and Central Metabolism to a Decrease of the Carbon Supply when Arabidopsis is Grown in Very Short Photoperiods Plant Cell & Environment (2009):.
[30] M. Stitt. Kontrolle des Pflanzenwachstums. In: Die Zukunft der Energie: Die Antwort der Wissenschaft Ed. by P. Gruss, F. Schüth., 2008. .
[31] B.B. Buchanan, W. Gruissem, W. G.. Biochemistry & Molecular Biology of Plants. Rockville: American Society of Plant Physiology, 2000
[32] K. Keegstra. Plant Cell Walls Plant Physiology 154: 483-486 (2010): 483-486.
[33] C. Somerville. Cellulose Synthesis in Higher Plants Annual Review of Cell and Developmental Biology 22: 53-78 (2006): 53-78.
[34] C. Somerville, S. Bauer, S. B., Brininstool S., B. S., G. Brininstool, G. B., Facette G., F. G., M. Facette. Toward a Systems Approach to Understanding Plant Cell Walls Science 306: 2206-2211 (2004): 2206-2211.
[35] J. Harholt, A. Suttangkakul, A. S.. Biosynthesis of Pectins Plant Physiology (2010):.
[36] H.V. Scheller, P. Ulvskov. Hemicelluloses Annual Review of Plant Biology 61: 263-289 (2010): 263-289.
[37] W. Boerjan, J. Ralph, J. R.. Lignin biosynthesis Annual Review of Plant Biology (2003):.
[38] C. Somerville, H. Youngs, H. Y., Taylor H., T. H.. Feedstocks for Lignocellulosic Biofuels Science 329: 790-792 (2010): 790-792.
[39] M.-M. Titirici, A. Thoma, A. T.. Back in the Black: Hydrothermal Carbonization of Plant Material as an Efficient Chemical Process to Treat the CO New Journal of Chemistry 31: 787-789 (2007): 787-789.
[40] E.A. Ainsworth, C. Beier, C. B., Calfapietra C., C. C., C. Calfapietra, C. C., Ceulemans C., C. C., R. Ceulemans, R. C., Durand-Tardif R., DT. R., M. Durand-Tardif, M. DT., Godbold M., G. M., D.L. Godbold, D. G., Hendrey D.L., H. D., G.R. Hendrey, G. H., Hickler G.R., H. G., T. Hickler, T. H., Kaduk T., K. T., J. Kaduk, J. K.. Next Generation of Elevated [CO Plant Cell and Environment 31: 1317-1324 (2008): 1317-1324.
[41]
[42] European Science Foundation (ESF). FACEing the Future: Planning the Next Generation of Elevated CO. Strasbourg: IREG, 2009
[43] United Nations Environment Programme (UNEP) () Towards Sustainable Production and Use of Resources: Assessing Biofuels.
[44]
[45]
[46] E. Sanchez-Monge () Taxonomia de las Magnoliofitas (Angiospermas) de Interés Agricola, con Excepción de las Aprovechamientos Exclusivamente Ornamental o Forestal.
[47] European Plant Science Organization (EPSO). European Plant Science: A Field of Opportunities Journal of Experimental Botany 56: 1699-1709 (2005): 1699-1709.
[48] F. Salamini. Hormones and the Green Revolution Science 302: 71-72 (2003): 71-72.
[49] J. Diamond. Guns, Germs and Steel. The Fates of Human Societies. London: Random House, 1997
[50] C. Pozzi, L. Rossini, L. R., Vecchietti L.. Gene and Genome Changes During Domestication of Cereals. In: Cereal Genomics Dordrecht, The Netherlands: Kluwer Academic Publishers, 2004. .
[51] S.P. Long, E.A. Ainsworth, E. A., Leakey E.A., L. E.. Food for Thought: Lower-Than-Expected Crop Yield Stimulations with Rising CO Science 312: 1918-1921 (2006): 1918-1921.
[52] S.P. Long, M.B. Jones, M. J.. Primary Productivity of Grass Ecosystems of the Tropics and Sub-Tropics. London: UNEP/Chapman & Hall, 1992
[53] C.V. Beale, S.P. Long. Can Perennial C4 Grasses Attain High Efficiencies of Radiant Energy Conversion in Cool Climates? Plant Cell & Environment 18: 641-650 (1995): 641-650.
[54] E.A. Heaton, F.G. Dohleman, F. D.. Meeting U.S. Biofuel Goals with Less Land: The Potential of Miscanthus Global Change Biology 14: 2000-2014 (2008): 2000-2014.
[55] C.L. Goodale, M.J. Apps, M. A., Birdsey M.J., B. M., R.A. Birdsey, R. B., Field R.A., F. R., C.B. Field, C. F., Heath C.B., H. C.. Forest Carbon Sinks in the Northern Hemisphere Ecological Application (2002):.
[56] J.E. Campbell, D.B. Lobell, D. L., Genova D.B.. The Global Potential of Bioenergy on Abandoned Agriculture Lands Environmental Science & Technology 42: 5791-5794 (2008): 5791-5794.
[57] P. Nobel. Environmental Biology of Agaves and Cacti. Cambridge: Cambridge University Press, 1988
[58] J.W. Purseglove. Tropical Crops—Monocotyledons. London, UK: Longman Ltd., 1972