Chapter structure
- 30.1 Introduction
- 30.2 Energy and Climate
- 30.3 The Scale of the Energy Challenge
- 30.4 Energy and Chemistry: EnerChem
- 30.5 Energy Scenarios
- 30.6 One Possible Target Scenario
- 30.7 Technical Summary
- 30.8 Global Aspects of Chemistry for Energy
- 30.9 Limiting Factors Within the Science
- 30.10 Global External Interfaces
- 30.11 Conclusions
- Acknowledgements
- References
- Footnotes
30.1 Introduction
The fifth oil price shock Doroodian and Boyd 2003; Kilian 2008; Herrera and Pesavento 2009 started from anticipated shortages in the availability and supply of the most visible energy carrier, and created intensive responses throughout society that have now prevailed for several years. Besides coping with the eco-political issues, for the first time we all feel that the present
This chapter intends to introduce the reader to a framework of challenges faced by science in approaching energy research.
Energy research is a broad interdisciplinary topic. A large variety of fundamental approaches are proposed and actively pursued in order to address the
This chapter attempts to elucidate the pivotal role of
30.2 Energy and Climate
It is now universally recognized that
In the essay part of the chapter, the term sustainability will also be used to describe a quality of research conduct. Energy science is sustainable for science only if validated understanding or reliable basic knowledge for technologies can be extracted from it. This hypothesis generates multiple consequences for individuals and the scientific community as a whole.
Even for purely chemical considerations, the systemic approach demands not only considerations about the efficiency, security and sustainability of the processes, but also about the availability of critical materials for the intended scale of use. In this respect, the application of noble metals or their compounds is a critical issue, exemplified by the use of Pt and Ru.
The already significant modification of the climate through CO2 emission calls for a most rapid reduction of further emissions.
30.3 The Scale of the Energy Challenge
To get a feeling for the dimension of energy requirements, the following
The purpose of this example, which does not include any growth in energy demand, is to illustrate the enormous challenge for any
On the other hand, it is necessary to focus to a certain extent on those issues7 that are critical for the near or foreseeable future. Many of them concern the
30.4 Energy and Chemistry: EnerChem
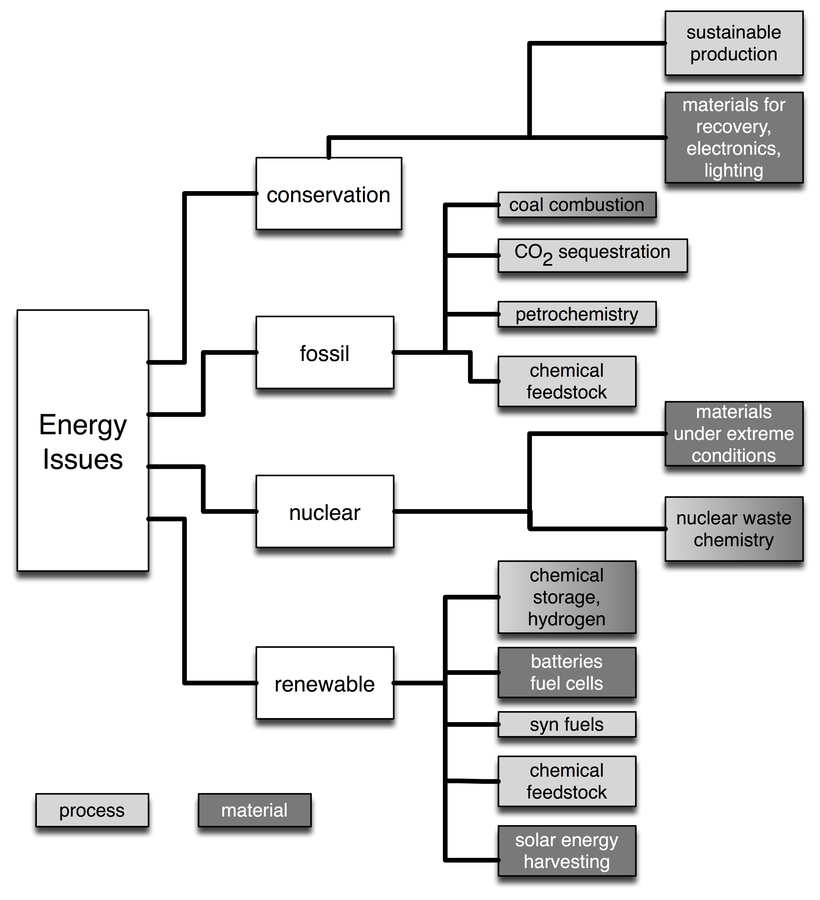
Fig. 30.1: EnerChem designates the multiple contributions of chemistry to the energy challenge. The shading indicates the main contribution from material (dark grey) and process (light grey) developments.
30.5 Energy Scenarios
In the present work, a different type of scenario is used. It is based on networks of technologies required to convert and store energy. Disregarding economic or political pressure, it describes the interaction of critical unit operations for providing energy to various non-related and non-coordinated applications (transportation, communication, production, domestic, retailing). The networks omit all physics-based components and are reduced to chemical processes. They assume the existence of grid systems for the exchange of electrical energy, for information exchange and for bulk material transport.
It is imperative to minimize greenhouse gas emissions as fast as possible in order to keep to a minimum the already unavoidable consequences of
Realistic energy scenarios take into account that, because the ownership of fossil and non-fossil energy carriers is not identical, competition will emerge with a strong pressure retarding the phasing out of fossil energy carriers. The timescales and intensity of this competitive situation will be determined largely by socio-political factors and by the awareness of the general public. The complex aggregation of non-scientific factors cannot be discussed in this work.
30.6 One Possible Target Scenario
Figure 30.2 presents a target scenario for an energy supply system based on regenerative primary energy coming from physical
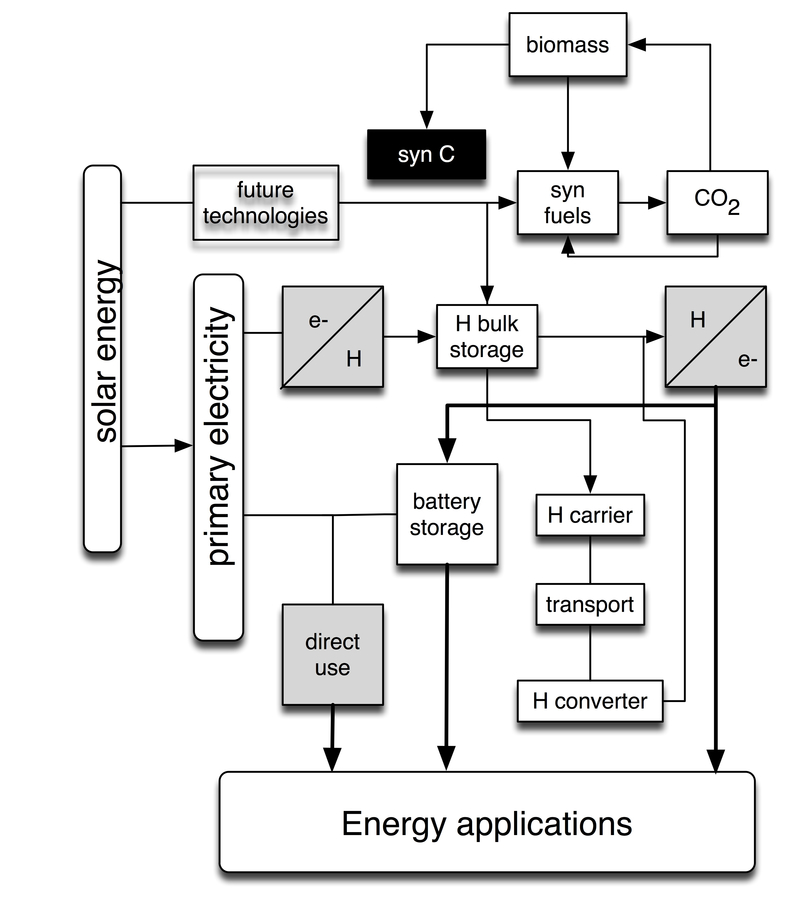
Fig. 30.2: A network of energy conversion processes requiring a minimal participation of carbon-based fuels that could be operative before fossil fuels are phased out. The shaded boxes designate families of chemical processes, whereas the unshaded boxes denote processes of physical origin. The boxes marked ‘syn fuels,’ ‘H carrier’ and ‘H converter’ indicate primarily catalytic reactions, whereas the other boxes designate other chemical processes in which catalysis may play a role.
The general structure of the scenario represented in Figure 30.2 concerns the flow of primary electricity from
Without effective and fast storage plus recovery systems there is no control over the temporal distribution of energy. Even with intelligent management strategies, the capacity of the electrical energy grid to regulate temporal differences between supply and demand is limited to about 15–20% of the load. With respect to the sustainability criteria, hydrogen is the suitable carrier, although other chemicals could become relevant in grid dimensions from solar-thermal processing Fletcher 1999; Lechon et.al. 2008, which is not considered here.
A great number of future energy consumers has no access to an electricity grid
30.6.1 Batteries
Only a small fraction of the primary electricity from solar sources (Figure 30.2) can be used directly; a larger portion may be stored in batteries Tarascon and Armand 2001; Wakihara 2001; Shaahid and El-Amin 2009, some of them operating in mobile applications such as cars. A substantial amount will have to be
Current research on batteries is largely phenomenological,14 resulting in a multiplicity of apparently competing “design” philosophies and still incremental improvements in performance. The necessary breakthrough to increase specific storage capacities will have to rely on an in-depth understanding of the complex interface processes of the charge-carrying chemical species (Li) during discharge and charge of the battery Breger et.al. 2007; Wontcheu et.al. 2008. Only few studies discuss the battery as a system of electrodes, electrolyte and membrane. As a result, the most studied process of Li metal storage is much better developed than the other equally necessary component operations of the battery. Furthermore, besides research on electrolytes, more study of the cathode is required: the need to store Li-ions and electrical charge carriers simultaneously has proved to be a bottleneck in the advancement of batteries. Many aspects of material transport through membranes, adsorption or intercalation into electrode solids and charge-exchange processes, are not yet understood on the necessary atomic level. In particular, the solid-solid reaction processes occurring during the charging and discharging of batteries Padhi et.al. 1997; Tarascon and Armand 2001; Pasero et.al. 2008 constitute an area of theoretical research15 concentrating on issues of defect dynamics, grain boundary chemistry and the reversibility of internal nanostructuring.
The design and control of these properties in functional storage materials require robust synthetic concepts and in-situ analytical tools rarely used so far Breger et.al. 2007; Agrawal et.al. 2008; McBreen 2009. The augmented use of solid-state NMR has given clear insight into the complexity Breger et.al. 2007; Wontcheu et.al. 2008 of the Li-ion battery system under operation. New in-situ tools are needed to provide experimental access to solid-fluid interfaces at ambient conditions. Synchrotron-based electron spectroscopy at tunable high energy and high resolution Salmeron and Schlögl 2008 can offer such information.16 Scalable synthetic concepts based upon composite materials such as nanostructured carbon systems Zhang et.al. 2008; Hu et.al. 2008; Hu et.al. 2009 will be carried further and extended to other functions, as chemists aspire to replace electrode materials such as Ru oxide, which are expensive or unavailable in bulk Armand et.al. 1985; Music et.al. 2002. Progress in this area depends on the capacity to handle the complexity of solid-liquid and solid-solid interfaces without present-day oversimplifications that prevent the simple translation of existing fundamental knowledge into functional systems.
30.6.2 Hydrogen
Hydrogen generation from water electrolysis19 is a fundamental challenge to
The combination of water electrolysis and primary electricity generation from PV Clarke et.al. 2009 or from wind has been studied quantitatively and reveals clearly that a combination of electrolysis with hydrogen storage is a technologically and scalable option far superior to other forms of electricity storage in terms of efficiency and storage capacity. The study using a PV array Clarke et.al. 2009 further demonstrated that such
Hydrogen is not a perfect energy carrier when it comes to mobile applications, which may be better served with a combination of mobile batteries and stationary electricity storage. Hydrogen is particularly unsafe for energy transfer over long distances. The
30.6.3 Carbon Dioxide
Alternative forms for hydrogen transport are methane or methanol Olah 2005; Olah et.al. 2009, which could be generated in dry sunny areas from CO2 and solar hydrogen in a bi-directional gas transport operation yielding the valuable by-product water. Here, substantial efforts in catalyst development and process design are still necessary Xu and Moulijn 1996; Aresta and Dibenedetto 2007 to reach grid-scale molecular conversions with acceptable energy losses (compared to those we face in the present energy supply system). CO2 may be considered as the raw material for a variety of uses, as shown in Figure 30.3.
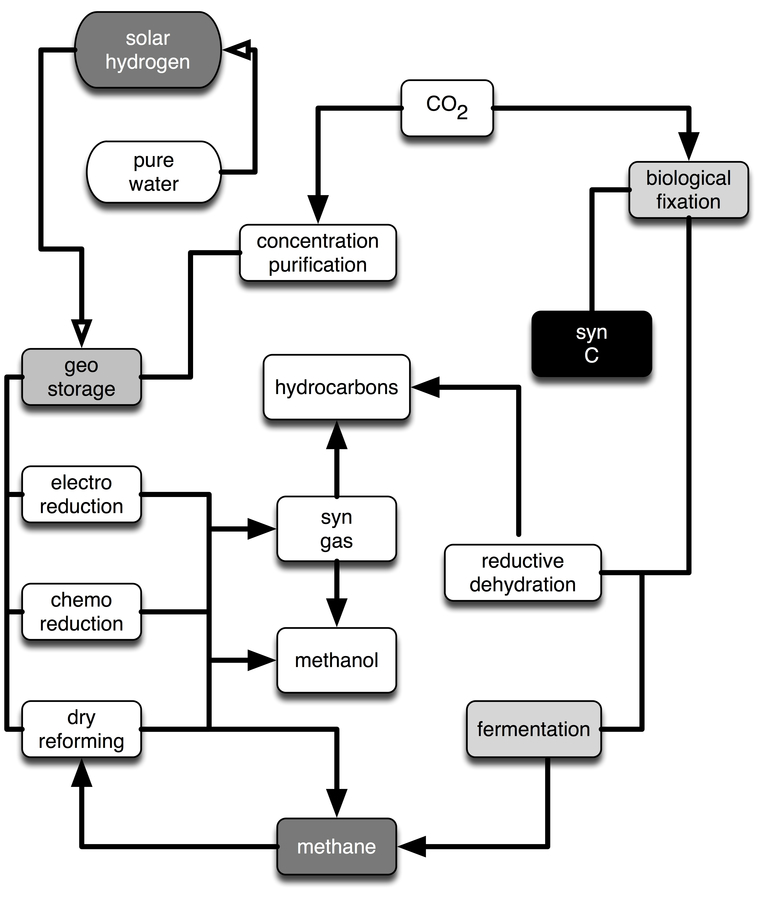
Fig. 30.3: Uses of CO2 as a raw material. Solar hydrogen is required in all conversion processes, including biological fixation. The boxes marked ‘electro reduction,’ ‘chemo reduction,’ ‘dry reforming,’ ‘syn gas’ and ‘reductive dehydration’ denote catalytic processes, the boxes ‘fermentation’ and ‘biological fixation’ stand for biological transformations. Methane is an intermediate product and energy carrier; the boxes ‘hydrocarbons’ and ‘methanol’ denote products for the
There are multiple uses of the greenhouse gas wherever abundant solar hydrogen allows the chemical transformation of CO2, or biological processes can harvest CO2 and the resulting biomass can be fermented or chemically refined. However, all of these uses are energetically unfavorable and require excessive
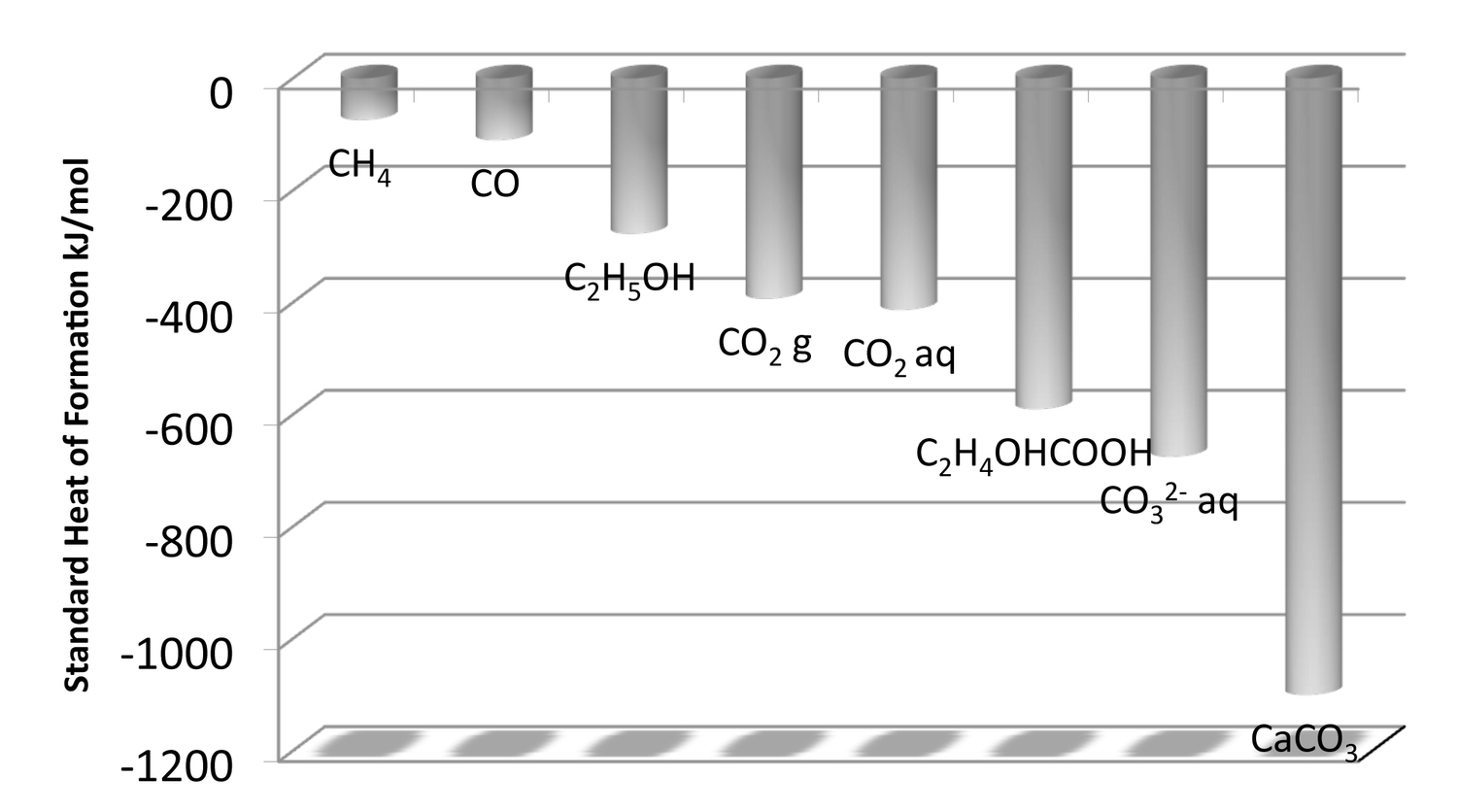
Fig. 30.4: Heat of formation data for some species related to CO2. The data was taken from Aresta and Dibenedetto 2007.
The reverse conversion process of hydrogen generation is the oxidation of hydrogen to water leading to electrical energy. This can be done rather conventionally by combustion in motors or turbines, or by fuel cell
A certain fraction of applications that require high energy density, such as planes, trucks and ships, will need synthetic fuels based on hydrocarbons. Using solar hydrogen and biological carbon sources, several process chains involving catalytic conversions that provide high quality synthetic fuels are feasible. The inclusion of biomass collection McKendry 2002a; McKendry 2002b; Islam et.al. 2004 balances the carbon dioxide emissions: alternatively, carbon dioxide, if collected at the source, can be hydrogenated with solar hydrogen Damen et.al. 2006 as a sequestration measure. In such a way, a sink for already emitted carbon can be constructed, and a bypass provided for the continuous emission of man-made CO2 to the natural deposition of carbon dioxide. All steps of the chemical transformations and the plant biology for the harvesting organisms need to be optimized substantially over existing technologies. Besides the challenging problem of CO2 hydrogenation on the global scale Xu and Moulijn 1996; Aresta and Dibenedetto 2007, the coupling of efficient plant biology to an effective chemical work-up of the biomass and the chemo-catalytic transformations require combined and concerted development.
This process chain is probably not suitable23 to generate the bulk of
30.6.4 Synthetic Fuels
Synthetic transportation fuels for applications that require high energy density can be generated chemically by a variety of methods, all of which are known in principle and are or have been operated on industrial scales. Figure 30.5 summarizes some options. Synthetic fuels are storage forms of solar hydrogen. The carbon part can come from fossil sources, from biomass or from CO2 as indicated in Figures 30.2 and 30.3. This part of the energy scenario is to a large extent proven
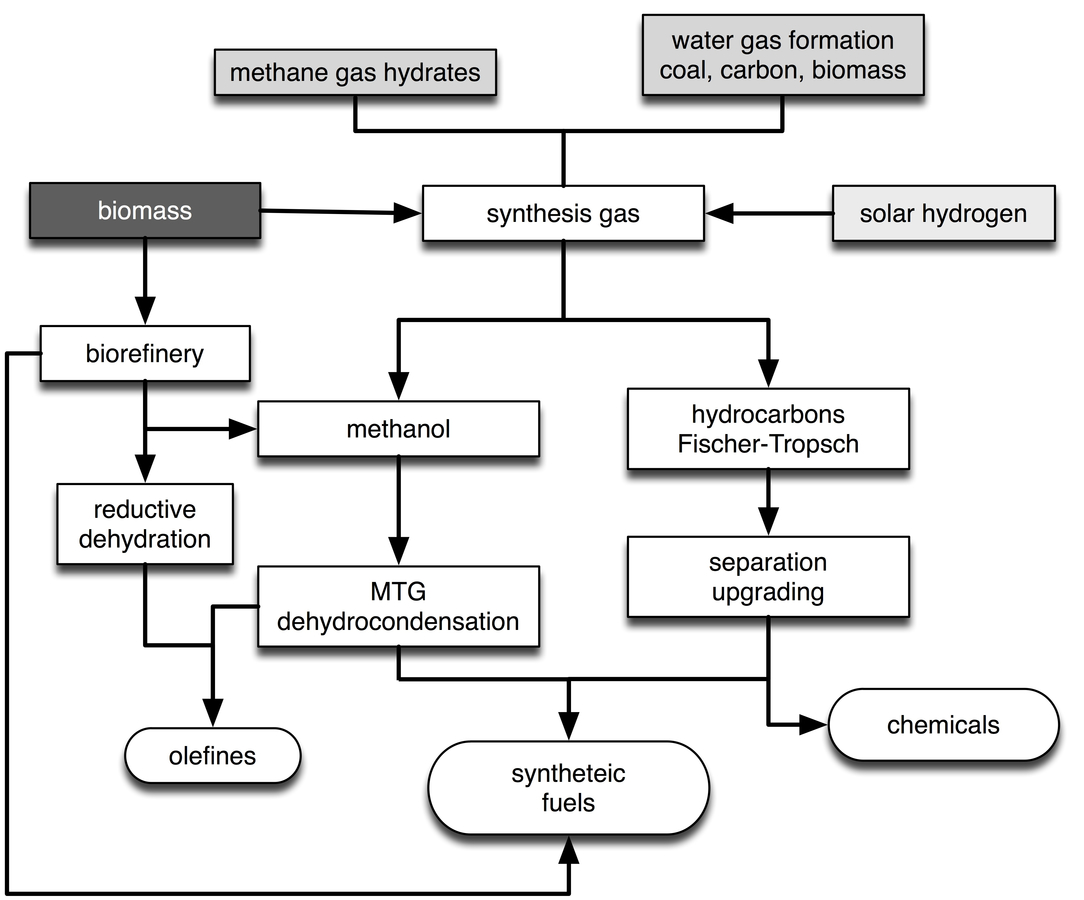
Fig. 30.5: Synthetic fuels can be generated by a wide variety of processes. Solar hydrogen is required and synthetic fuels are thus a storage form of hydrogen. The carbon part can be taken from fossil sources or from CO2 as shown in Figure 30.2.
Biological fixation of CO2 offers the attractive option of depositing carbon dioxide as inorganic carbon after harvesting through biomass. In this way, a sink for CO2 emissions is created, which can be operated such that no extra energy besides that stored in the biomass is utilized to generate “synC.” This product is a largely aliphatic carbon polymer with a substantial oxygen content Titirici et.al. 2007; Titirici et.al. 2008; Paraknowitsch et.al. 2009. Its structure is fundamentally different from that of coal24 The material is thus not suitable as a novel fossil energy carrier substitute Longwell et.al. 1995; Van Heek 2000, termed “artificial coal” or “accelerated coal.” It may be used to regenerate the soil losses from carbon Mader et.al. 2002; Lal 2004 and thus help enhance agricultural productivity. Additional non-energy uses of this carbon feedstock are conceivable Haenel 1992; Song and Schobert 1996; Schobert and Song 2002, but much additional work will be required to tailor the structure according to potential uses. A novel field for carbon
30.7 Technical Summary
The three disciplinary sub-forms of heterogeneous, homogeneous and biological catalysis bear a pivotal responsibility in solving the challenge of converting small molecules. Clearly, the present efforts in “energy catalysis” are still insufficient. The frequent discussion of potential energy conversion processes in terms of the technological hurdles of “inefficiency” should not be conducted in the conventional phenomenological manner, by recurring to qualitative concepts. A fundamental approach is needed, respecting the unity of catalysts and reactant as a system with multiple feedback relations in between Norskov et.al. 2002, which could be derived using the examples of converting energy storage molecules. From there, breakthroughs in improving the present technology levels can be expected.
Chemical energy research must expand as broadly as possible in order to capture many possible solutions. The research strategy may be structured in the long-term “discovery searches” discussed elsewhere,25 and in activities driven by the shorter-term intention to facilitate the transition from pure fossil into a fossil-plus-renewable energy
Converting primary electricity into chemical energy and storing
The immediacy and urgency for finding a large-scale solution makes it tempting to prioritize the various concepts. However, it seems inappropriate to favor or disfavor any of these strategies at present, while we have still insufficient insight into the structure of future energy supply networks. The scientific exploration of all of the concepts must have priority before decisions can be taken outside the scientific community in favor of or against any of these concepts.
As there will always be the need for non-electrical energy, it is further useful to study direct chemical solar energy harvesting through high-temperature processes and solar-thermochemistry Fletcher 1999; Kalogirou 2004. These processes and their chemical implications are not covered here. Solar-thermal power generation requires energy absorbers with unprecedented functional properties. Solar-chemical processes present major challenges to solid-state chemistry, as ways must be found to control the kinetic issues of solid-gas transformations.
The present approach stands in some contrast to the more radical suggestion of using chemistry to build an artificial photosynthesis device26 as a single-step Lewis 2006 process. This most challenging approach may be an element in later-generation energy supply networks Grubler et.al. 1999. The enormous hurdles to developing, optimizing and implementing such a device into a
The use of hydrogen has been the subject of multiple and controversial discussions in the past Barreto et.al. 2003; Muradov and Veziroglu 2008. Acknowledging the arguments and taking into account the critical need to reduce our carbon footprint, we suggest using hydrogen as a universal energy storage molecule as soon as possible. This does not imply using small mobile hydrogen storage devices Schlapbach and Zuttel 2001, but rather buffering the grid against fluctuations using stationary installations Christie et.al. 2000. Transportation energy, a key target of the concept of a
Biomass-based
30.8 Global Aspects of Chemistry for Energy
This surprising knowledge gap is due in part to lacking technology demands, and in part to the present socio-economic system’s inertia in responding to the challenge. The global nature of this system, outlined in several contributions to this volume, creates the global dimension of the
The following section will elaborate on some factors affecting the necessary rapid adaptation and change in our energy supply scenario. These factors are illustrated from the point of view of a scientist. We identify factors inherent in science and other factors from outside the field that represent boundary conditions of energy research. The term “science” is understood here as describing a global scientific community of researchers active in energy research and more closely in EnerChem
The origin of the
30.9 Limiting Factors Within the Science
30.9.1 Lack of Awareness
Many
30.9.2 Lack of Sustainability
In energy research, sustainability has to address both the solutions and the research effort. All chemical
Energy research is not sustainable unless it follows an initial idea, via laboratory feasibility studies, to a level of execution at which life-cycle considerations can assess the idea’s usefulness on a larger scale. What is at stake here is the complex issue of the
The evolution of new dimensions of
A critical scientist’s estimation of the extent to which a hypothetical solution to an energy problem may be sustainable is often considered “non-scientific” or “applied” and hence not highly valued. This prejudice may be of some value with respect to predictions concerning the possibilities of
30.9.3 The Role of Theoretical Science
The arguments made in the preceding section open the discourse about the role of theoretical science in the energy arena. From the view of augmenting our fundamental understanding, no project can be rejected, provided that it is conducted with the scientific rigor necessary to allow insights to be derived from it.
One trend is the belief in a phenomenological search for solutions. Besides many dead-ends, several creative and new concepts may arise from this approach. However, they do require serious and rigorous investigation after their identification. Yet the global practice is to initiate the founding of start-up companies and attempt to commercialize solutions without having the necessary scientific understanding. The other trend is that nothing follows upon an initial report of a new discovery and the science “caravan” moves on.
This practice wastes many resources in academia and in the economy. Moreover, it has the strong negative effect of discouraging whole lines of solution strategies once the first element fails to be realized commercially, or to set a research trend thanks to rapid and simple reproduction. The approach further creates a spirit of exaggerated expectations by societal forces, which is anything but justified in view of the magnitude of the
It is extremely important, however, to recognize from the technical part of this chapter that the
The fundamental aspects of energy science and the long timescale of its operation also entail the need to develop suitable curricula to educate energy science specialists in several research fields inside and outside of chemistry.28 This has been realized in some engineering disciplines, but is not so common in traditional basic sciences.
30.9.4 Interdisciplinarity
The lack of a suitable technical language shared by neighboring disciplines. This deficit inhibits the first contacts between scientists, creates prejudices and builds hurdles to communication.
The lack of basic knowledge about best practices between disciplines and the limitations to facilitating interdisciplinary discourse through the transfer of knowledge. A typical example is a lack of mathematical training hampering the concise exchange and evaluation of data.
The disharmony of practices by graduate and undergraduate students between disciplines as well as between countries in frequently needed international
The dominant role of the pressure to seek immediate
This incomplete list looks quite negative in light of the clear trend of global science to foster and encourage interdisciplinary research. Against this stands merely the time horizon to bring researchers of different national and disciplinary backgrounds together and let them develop the necessary common working ground beyond superficial demonstrations. Such forced interdisciplinary activity is not suitable to bring about truly new science and, due to its bureaucratic overhead, can prevent even
30.9.5 Scholarly Coordination
Some areas of science are traditionally well coordinated on a global basis.
It is thus not expected that traditional chemistry will take the lead to better organize the research efforts in energy sciences from within the community of practitioners. It is more likely that a new community of “energy chemistry” may form from the boundary regions of disciplinary chemistry. This new community may then practice self-organization in a way that includes coordinated efforts directed toward internal projects as well as toward interaction with the external parts of the scientific community.
It is not advocated that external bodies such as funding agencies or science planners occupy this niche of organization of scientific activity. Neither is the sometimes inadequate presentation of normal scholarly discourse to the general public (as recently with the IPCC) helpful in a situation where society expects from its many scientists a constructive and serious attempt to solve this major challenge. Any moves presenting individual views or even profiling measures to the public are fatal to the aspired-to and essential self-organization of energy science.
30.10 Global External Interfaces
We assume that the ability of chemistry to deliver its critical contributions is also limited by factors outside the scientific community. These factors are not independent from the community, as there are multiple transactions across the boundary. But because decisions to moderate the adverse influences cannot come from within the community these factors are classified as external.
30.10.1 Lack of Independence
Energy science is an expensive operation requiring billions of dollars for its effective operation. There is great public support for energy science, at least within the usual five-year life cycle of standard funding. Indeed, the fact that many energy science centers have been created worldwide documents that the long-term character of the challenge has been recognized. This should give science the necessary independence to develop its strategic views and to bring about reliable solutions based upon rigorous understanding.
This ideal view is distorted by the strong influence of economic factors. Both individual researchers and funding bodies readily follow the argument that a given research idea has to be evaluated on the grounds of real or hypothetical economic feasibility against the backdrop of the present
The energy economy is not a free market, but highly regulated by local and international political settings. Global enterprises, cartels of production, subsidies on energy use and the fact that no price has to be paid for producing CO2 are strong factors that make energy prices dependent on politics rather than on non-speculative market forces. The accuracy to which an economic break-even of a technology can be judged is thus limited and may be obsolete when political boundary conditions change. The debate about the value of photovoltaic devices in Europe is a timely example for such a critical influence of “economic” considerations.
Economic factors directly influence decisions to fund energy projects. The debate about electro-mobility and required advanced
Economic factors also affect the development of regionally adapted solutions. Global trade and the massive trend to outsource production from high-level societies into emerging economies exports not only labor but also
Economic factors stabilize the existing infrastructure and thus tend to delay the market penetration of novel
30.10.2 Dissemination and Open Access
If
30.10.3 Relation to Politics
The history of chemical accidents in production is a vivid example of how inadequate communication can ruin the efforts of a whole science. Today, chemistry has a bad reputation in society and politics stemming from the false image that it has denied risks and scrimped on safety measures. This reputation represents another hurdle for dialogue between science and politics. The chemical science community is not as attractive as other branches of science and thus not so present in the small budget of attention that politics tends to share with all sciences.
The complex relation of chemical sciences with politics in general has an adverse effect on the flow of information, with the consequence that incorrect views spread easily within the political debate. This does not enhance the influence of science versus economic interests when it comes to strategic decisions or the design of funding programs. These deficits are less a challenge for Anglo-Americans, with their different approach to discourse and lobbying than in Europe. In Asia, politics pays a great deal of attention to the energy challenge, and despite the strong influence of economy, science organizations also enjoy substantial influence in the debate. It can be stated that inadequate self-organization, paired with the issue of chemistry’s reputation, results in a deficit in the dialogue with politics. This is less a problem for issues affecting the traditional
30.10.4 Chemistry and Society
The demand for energy is a global issue. However, its origin and the societal context in which it is projected are local and quite diverse.
Chemistry may have to learn that these diverse needs do exist in addition to local perceptions. The local view of an individual scientist in a local society finds its reflection in controversial views on approaches and solutions within the global community. Much of this discussion is not fruitful and hinders the evolution of our understanding. It further spills out to society, where it is often abused for political debates not intended by the scientific discourse. It would be a great achievement if energy researchers would become open enough to improve contact with their local society and then reflect their experiences upon the global view of consolidated international results. These contacts should be bi-directional in the sense that both parties exchange information. Societal concerns are serious boundary conditions to science and technology as illustrated by the example of
The energy issue demands from its researchers a substantial dose of
The societal context of energy science demands critical competence by the researcher to perform a rudimentary assessment of a product life cycle and of process sustainability. Both criteria are better indications for priorization of resources than the expected distance to market. The central responsibility of the energy researcher is, however, to remain driven by curiosity and rigor in practical actions. Missed opportunities are the greatest loss that society can suffer from the work of science. This is true globally, calling for moderation in the speed of the race toward
It is superficial to analyze the behavior of science and society within a single point of view. Many activities support energy science both within the community and within society as a whole. The global operation of science greatly accelerates these activities, leading to similar thoughts and concepts. It remains the responsibility of individuals to use the positive momentum of global science and combine it with the local character of challenges and required solutions.
30.11 Conclusions
Most efforts to cope with the challenge are directed towards enabling strategies put forward by the
Science would have to play a pivotal role in this situation. It is the only institution that can provide a basis of facts free of political or economic interests. It would have to deliver reliable information for decision makers in industry and politics. Moreover, it would have to construct a sustainable basis for creating the technology options required to make responsible strategic decisions. Such decisions are frequently demanded within short timescales. The need to sustain the value of capital investments and the target of minimizing the consequences of
The analyzing and modeling part of climate science has made an impressive effort to coordinate its actions under the umbrella of the IPCC, which may still need improvement.33 This truly global science organization may serve as a prototype for other energy sciences that have to provide the solution strategies and serve those who bear the responsibility for implementation. In these areas of science, encompassing both natural and social disciplines, globally responsible and sustainable operation is still lacking, resulting in disorientation, duplication of activities and overall inefficiency. The present chapter does not advocate a gigantic coordinating structure. Instead, it calls for a global network to provide a blueprint for a hierarchical structure of energy science. This hierarchy would be multi-dimensional in traditional disciplines and in the distribution between theoretical and applied research. In such a strategy the hurdles of lacking communication,
Energy is an area where science, society and economy meet in a critical discourse.
Acknowledgements
The author is grateful to all members of the EnerChem consortium within the Max Planck Society for many fruitful discussions that helped to shape these ideas.
References
Agrawal, R. C., A. Chandra, A. C., Bhatt A. (2008). Investigations on Ion Transport Properties of and Battery Discharge Characteristic Studies on Hot-Pressed Ag + -Ion-conducting Nano-Composite Polymer Electrolytes: (1-x) [90PEO: 10AgNO. New Journal of Physics
Alanne, K., A. Saari (2006). Distributed Energy Generation and Sustainable Development. Renewable and Sustainable Energy Reviews 10(6): 539-558
Alstrum-Acevedo, J. H., M. K. Brennaman, M.K. B. (2005). Chemical Approaches to Artificial Photosynthesis.. Inorganic Chemistry 44(20): 6802-6827
Antelman, K. (2004). Do Open-Access Articles Have a Greater Research Impact?. College & Research Libraries 65(5): 372-382
Archer, D., V. Brovkin (2008). The Millennial Atmospheric Lifetime of Anthropogenic CO. Climatic Change 90(3): 283-297
Archer, M. D., J. R. Bolton (1990). Requirements for Ideal Performance of Photochemical and Photovoltaic Solar-Energy Converters. Journal of Physical Chemistry 94(21): 8028-8036
Aresta, M., A. Dibenedetto (2007). Utilisation of CO. Dalton Transactions 28: 2975-2992
Arico, A. S., P. Bruce, P. B., Scrosati P., S. P. (2005). Nanostructured Materials for Advanced Energy Conversion and Storage Devices. Nature Materials 4: 366-377
Armand, M., F. Dalard, F. D., Deroo F. (1985). Modeling the Voltametric Study of Intercalation in a Host Structure: Application to Lithium Intercalation in RuO. Solid State Ionics 15(3): 205-210
Armbruster, C. (2007). Moving out of Oldenbourg's Long Shadow: What is the Future for Society Publishing?. Learned Publishing 20: 259-266
Aroutiounian, V. M., V. M. Arakelyan, V.M. A. (2005). Metal Oxide Photoelectrodes for Hydrogen Generation Using Solar Radiation-driven Water Splitting. Solar Energy 78(5): 581-592
Asif, M., T. Muneer (2007). Energy Supply, its Demand and Security Issues for Developed and Emerging Economies. Renewable and Sustainable Energy Reviews 11(7): 1388-1413
Bak, T., J. Nowotny, J. N., Rekas J. (2002). Photo-Electrochemical Hydrogen Generation from Water Using Solar Energy: Materials-related Aspects. International Journal of Hydrogen Energy 27(10): 991-1022
Balaya, P. (2008). Size Effects and Nanostructured Materials for Energy Applications. Energy and Environmental Science 1(6): 645-654
Barber, James (2009). Photosynthetic Energy Conversion: Natural and Artificial. Chemical Society Reviews 38(1): 185-196
Barreto, L., A. Makihira, A. M. (2003). The Hydrogen Economy in the 21st Century: A Sustainable Development Scenario. International Journal of Hydrogen Energy 28(3): 267-284
Beccali, M., M. Cellura, M. C., Brano M. (2004). Forecasting Daily Urban Electric Load Profiles Using Artificial Neural Networks. Energy Conversion and Management 45: 2879-2900
Berntsen, T., J. Fuglestvedt, J. F., Myhre J., M. J. (2006). Abatement of Greenhouse Gases: Does Location Matter?. Climatic Change 74(4): 377-411
Best-Waldhober, M., Daamen de, D. d. (2009). Informed and Uninformed Public Opinions on CO. International Journal of Greenhouse Gas Control 3: 322-332
Bockris, J. O., E. C. Potter (1952). The Mechanism of Hydrogen Evolution at Nickel Cathodes in Aqueous Solutions. Journal of Chemical Physics 20(4): 614-628
Bolton, J. R., D. O. Hall (1979). Photochemical Conversion and Storage of Solar Energy. Annual Review of Energy 4: 353-401
Breger, J., K. Kang, K. K., Cabana K., C. K. (2007). NMR, PDF and RMC Study of the Positive Electrode Material Li(Ni. Journal of Materials Chemistry 17(30): 3167-3174
Chan, Hong-Wei, Jenq-Gong Duh, JG. D. (2006). In situ Synchrotron X-Ray Diffraction Investigations of LiCu. Journal of the Electrochemical Society 153(8): A1533-1538
Charles, D. (2009). ENERGY: Renewables Test IQ of the Grid. Science 324(5924): 172-175
Cheng, C. H., J. Lehmann, J. L., Thies J., T. J. (2006). Oxidation of Black Carbon by Biotic and Abiotic Processes. Organic Geochemistry 37(11): 1477-1488
Christensen, C. H., T. Johannessen, T. J., Sorensen T. (2006). Towards an Ammonia-mediated Hydrogen Economy?. Catalysis Today 111(1–2): 140-144
Christensen, C. H., R. Z. Sorensen, R.Z. S., Johannessen R. Z., J. R.Z., T. Johannessen, T. J., Quaade T. (2005). Metal Ammine Complexes for Hydrogen Storage. Journal of Materials Chemistry 15(38): 4106-4108
Christie, R. D., B. F. Wollenberg, B.F. W. (2000). Transmission Management in the Deregulated Environment. Proceedings of the IEEE 88(2): 170-195
Claassen, P. A. M., J. B. Lier, J.B. L., van J. B., v. J.B., Contreras van, C. v., A. M. L. Contreras (1999). Utilisation of Biomass for the Supply of Energy Carriers. Applied Microbiology and Biotechnology 52: 741-755
Clark, J. H., F. E. I. Deswarte, F.E.I. D. (2009). The Integration of Green Chemistry into Future Biorefineries. Biofuels Bioproducts & Biorefining-Biofpr 3: 72-90
Clarke, R. E., S. Giddey, S. G., Ciacchi S., C. S., F. T. Ciacchi (2009). Direct Coupling of an Electrolyser to a Solar PV System for Generating Hydrogen. International Journal of Hydrogen Energy 34(6): 2531-2542
Conte, M., G. Budroni, G. B., Bartley G., B. G., J. K. Bartley, J.K. B., Taylor J. K., T. J.K., S. H. Taylor, S.H. T. (2006). Chemically Induced Fast Solid-State Transitions of Omega-VOPO. Science 313(5791): 1270-1273
Conway, B. E., J. O. Bockris (1957). Electrolytic Hydrogen Evolution Kinetics and its Relation to the Electronic and Adsorptive Properties of the Metal. Journal of Chemical Physics 26(3): 532-541
Cosmi, C., S. Di Leo, S. D.L., Loperte S., L. S., S. Loperte, S. L. (2009). A Model for Representing the Italian Energy System: The NEEDS-TIMES Experience. Renewable and Sustainable Energy Reviews 13(4): 763-776
Croce, F., G. B. Appetecchi, G.B. A., Persi G. B. (1998). Nanocomposite Polymer Electrolytes for Lithium Batteries. Nature 394(6692): 456-458
Damen, K., M. Troost, M. T., van M. (2006). A Comparison of Electricity and Hydrogen Production Systems with CO. Progress in Energy and Combustion Science 32: 215-246
Deshmukh, M. K., S. S. Deshmukh (2008). Modeling of Hybrid Renewable Energy Systems. Renewable and Sustainable Energy Reviews 12(1): 235-249
Doroodian, K., R. Boyd (2003). The Linkage Between Oil Price Shocks and Economic Growth with Inflation in the Presence of Technological Advances: A CGE Model. Energy Policy 31(10): 989-1006
Egan, A. (2009). A Moral Climate: The Ethics of Global Warming. Theological Studies 70(2): 491-493
Ertl, G., H. J. Freund (1999). Catalysis and Surface Science. Pysics Today 52(1): 32-38
Fierro, J. L. G. (1993). Catalysis in C₁ Chemistry: Future and Prospect. Catalysis Letters 22(1–2): 67-91
Fischer, M. (1986). Review of Hydrogen-Production with Photovoltaic Electrolysis Systems. International Journal of Hydrogen Energy 11(8): 495-501
Fisher, G. B., J. L. Gland, J.L. G. (1982). The Spectroscopic Observation of Water Formation. Journal of Vacuum Science and Technology 20(3): 518-521
Fletcher, E. A. (1999). Solarthermal and Solar Quasi-Electrolytic Processing and Separations: Zinc from Zinc Oxide as an Example. Industrial and Engineering Chemistry Research 38(6): 2275-2282
Friedmann, S. J. (2007). Geological Carbon Dioxide Sequestration. Elements 3(3): 179-184
Gao, Y., J. R. Dahn (1996). Synthesis and Characterization of Li. Journal of the Electrochemical Society 143(1): 100-114
Ghanadan, R., J. G. Koomey (2005). Using Energy Scenarios to Explore Alternative Energy Pathways in California. Energy Policy 33(9): 1117-1142
Gooch, D. J. (2000). Materials Issues in Renewable Energy Power Generation. International Materials Reviews 45(1): 1-14
Gorbaty, M. L. (1994). Prominent Frontiers of Coal Science: Past, Present and Future. Fuel 73(12): 1819-1828
Gratzel, M. (1981). Artificial Photosynthesis: Water Cleavage into Hydrogen and Oxygen by Visible Light. Accounts of Chemical Research 14(12): 376-384
Greeley, J., T. F. Jaramillo, T.F. J., Bonde T. F., B. T.F. (2006). Computational High-Throughput Screening of Electrocatalytic Materials for Hydrogen Evolution. Nature Materials 5(11): 909-913
Greeley, J., J. K. Norskov (2007). Large-scale, Density Functional Theory-based Screening of Alloys for Hydrogen Evolution. Surface Science 601(6): 1590-1598
Grimsdale, A.C., K. Mullen (2005). The Chemistry of Organic Nanomaterials. Angewandte Chemie-International Edition 44: 5592-5629
Grubler, A., N. Nakicenovic, N. N. (1999). Dynamics of Energy Technologies and Global Change. Energy Policy 27(5): 247-280
Haenel, M. W. (1992). Recent Progress in Coal Structure Research. Fuel 71(11): 1211-1223
Haile, S. M. (2003). Fuel Cell Materials and Components. Acta Materialia 51(19): 5981-6000
Hall, D. O. (1978). Solar Energy Conversion Through Biology: Could it Be a Practical Energy Source?. Fuel 57(6): 322-333
Havecker, M., R. W. Mayer, R.W. M., Knop-Gericke R. W., KG. R.W., A. Knop-Gericke, A. KG., Bluhm A., B. A., H. Bluhm, H. B., Kleimenov H., K. H., E. Kleimenov, E. K. (2003). In situ Investigation of the Nature of the Active Surface of a Vanadyl Pyrophosphate Catalyst During n-Butane Oxidation to Maleic Anhydride. Journal of Physical Chemistry B 107(19): 4587-4596
Heller, A. (1981). Conversion of Sunlight into Electrical Power and Photoassisted Electrolysis of Water in Photoelectrochemical Cells. Accounts of Chemical Research 14(5): 154-162
Hellman, A., E. J. Baerends, E.J. B., Biczysko E. J., B. E.J., M. Biczysko, M. B., Bligaard M., B. M., T. Bligaard, T. B., Christensen T., C. T., C. H. Christensen, C.H. C., Clary C. H., C. C.H., D. C. Clary, D.C. C., Dahl D. C. (2006). Predicting Catalysis: Understanding Ammonia Synthesis from First-Principles Calculations. Journal of Physical Chemistry B 110(36): 17719-17735
Herbert, G. M. J., S. Iniyan, S. I., Sreevalsan S. (2007). A Review of Wind Energy Technologies. Renewable and Sustainable Energy Reviews 11(6): 1117-1145
Herrera, A. M., E. Pesavento (2009). Oil Price Shocks, Systematic Monetary Policy, and the “Great Moderation”. Macroeconomic Dynamics 13(1): 107-137
Hofmann, D. J., J. H. Butler, J.H. B. (2009). A New Look at Atmospheric Carbon Dioxide. Atmospheric Environment 43(12): 2084-2086
Hu, Y. S., R. Demir-Cakan, R. DC., Titirici R., T. R., M. M. Titirici, M.M. T. (2008). Superior Storage Performance of a Si@SiOx/C Nanocomposite as Anode Material for Lithium-Ion Batteries. Angewandte Chemie International Edition 47(9): 1645-1649
Hu, Y. S., X. Liu, X. L., Muller X., M. X., J. O. Muller (2009). Synthesis and Electrode Performance of Nanostructured V. Angewandte Chemie International Edition 48(1): 210-214
Islam, M., A. Fartaj, A. F. (2004). Current Utilization and Future Prospects of Emerging Renewable Energy Applications in Canada. Renewable and Sustainable Energy Reviews 8(6): 493-519
Jamnik, J., J. Maier (2003). Nanocrystallinity Effects in Lithium Battery Materials: Aspects of Nano-Ionics. Part IV. Physical Chemistry Chemical Physics 5(23): 5215-5220
Jaramillo, T. F., K. P. Jorgensen, K.P. J., Bonde K. P., B. K.P., J. Bonde (2007). Identification of Active Edge Sites for Electrochemical H. Science 317(5834): 100-102
Kalogirou, S. A. (2004). Solar Thermal Collectors and Applications. Progress in Energy and Combustion Science 30(3): 231-295
Kanan, M. W., D. G. Nocera (2008). In situ Formation of an Oxygen-Evolving Catalyst in Neutral Water Containing Phosphate and Co . Science 321(5892): 1072-1075
Kanan, M. W., Y. Surendranath, Y. S. (2009). Cobalt-Phosphate Oxygen-Evolving Compound. Chemical Society Reviews 38(1): 109-114
Kaundinya, D. P., P. Balachandra, P. B. (2009). Grid-connected versus Stand-Alone Energy Systems for Decentralized Power: A Review of Literature. Renewable and Sustainable Energy Reviews 13(8): 2041-2050
Ketzer, J. M., R. Iglesias, R. I., Einloft R., E. R., S. Einloft (2009). Water-Rock-CO. Applied Geochemistry 24(5): 760-767
Khan, N., Z. Saleem, Z. S. (2008). Review of Natural Energy Sources and Global Power Needs. Renewable and Sustainable Energy Reviews 12(7): 1959-1973
Kilian, L. (2008). The Economic Effects of Energy Price Shocks. Journal of Economic Literature 46(4): 871-909
Klerke, A., C. H. Christensen, C.H. C., Norskov C. H. (2008). Ammonia for Hydrogen Storage: Challenges and Opportunities. Journal of Materials Chemistry 18(20): 2304-2310
Knop-Gericke, A., E. Kleimenov, E. K., Havecker E., H. E., M. Havecker, M. H., Blume M., B. M., R. Blume, R. B., Teschner R., T. R., D. Teschner, D. T. (2009). X-Ray Photoelectron Spectroscopy for Investigation of Heterogeneous Catalytic Processes. Advances in Catalysis 52: 213-272
Kodama, T. (2003). High-Temperature Solar Chemistry for Converting Solar Heat to Chemical Fuels. Progress in Energy and Combustion Science 29(6): 567-597
Kothari, R., D. Buddhi, D. B. (2008). Comparison of Environmental and Economic Aspects of Various Hydrogen Production Methods. Renewable and Sustainable Energy Reviews 12(2): 553-563
Kunkes, E. L., D. A. Simonetti, D.A. S., West D. A., W. D.A., R. M. West (2008). Catalytic Conversion of Biomass to Monofunctional Hydrocarbons and Targeted Liquid-Fuel Classes. Science 322: 417-421
Lal, R. (2004). Soil Carbon Sequestration to Mitigate Climate Change. Geoderma 123(1–2): 1-22
Law, C. K., O. C. Kwon (2004). Effects of Hydrocarbon Substitution on Atmospheric Hydrogen-Air Flame Propagation. International Journal of Hydrogen Energy 29(8): 867-879
Lechon, Y., C. Rua, C. R. (2008). Life Cycle Environmental Impacts of Electricity Production by Solarthermal Power Plants in Spain. Journal of Solar Energy Engineering-Transactions of the Asme
Leitner, W. (1996). The Coordination Chemistry of Carbon Dioxide and its Relevance for Catalysis: A Critical Survey. Coordination Chemistry Reviews 153: 257-284
LeNeveu, D. M. (2008). CQUESTRA, a Risk and Performance Assessment Code for Geological Sequestration of Carbon Dioxide. Energy Conversion and Management 49(1): 32-46
Leon, C. P., Frias-Ferrer de, FF. d., A. Frias-Ferrer, A. FF. (2006). Redox Flow Cells for Energy Conversion. Journal of Power Sources 160: 716-732
Lewis, Rhodri (2006). From Athens to Elsinore: The Early Modern Art of Memory, Reconsidered. Preprint 319. Berlin: Max Planck Institute for the History of Science.
Li, Xin-Yu, Hai-Ping Tang (2006). Carbon Sequestration: Manners Suitable for Carbon Trade in China and Function of Terrestrial Vegetation. Zhiwu Shengtai Xuebao 30(2): 200-209
Liu, Y., Y. Zhang, Y. Z., Wang Y. (2007). Efficient Conversion of Carbon Dioxide to Methanol Using Copper Catalyst by a New Low-Temperature Hydrogenation Process. Chemistry Letters 36: 1182-1183
Longwell, J. P., E. S. Rubin, E.S. R. (1995). Coal: Energy for the Future. Progress in Energy and Combustion Science 21(4): 269-360
Lovegrove, K. M. (1996). High Pressure Ammonia Dissociation Experiments for Solar Energy Transport and Storage. International Journal of Energy Research 20(11): 965-978
Lubitz, W., E. J. Reijerse, E.J. R. (2008). Solar Water-Splitting into H. Energy and Environmental Science 1(1): 15-31
Mader, P., A. Fliessbach, A. F., Dubois A., D. A., D. Dubois (2002). Soil Fertility and Biodiversity in Organic Farming. Science 296(5573): 1694-1697
Manthiram, A., A. V. Murugan, A.V. M., Sarkar A. V. (2008). Nanostructured Electrode Materials for Electrochemical Energy Storage and Conversion. Energy and Environmental Science 1(6): 621-638
Marsh, H., J. L. Figueiredo, J.L. F. (1986). Structure in Carbons. In: Carbon and Coal Gasification: Science and Technology Dordrecht: Martinus Nijhoff Publishers 27-56
Martin, H. B., A. Argoitia, A. A., Landau A., L. A. (1996). Hydrogen and Oxygen Evolution on Boron-doped Diamond Electrodes. Journal of the Electrochemical Society 143(6): L133-L136
McBreen, J. (2009). The Application of Synchrotron Techniques to the Study of Lithium-Ion Batteries. Journal of Solid State Electrochemistry 13(7): 1051-1061
McKendry, P. (2002a). Energy Production from Biomass (part 1): Overview of Biomass. Bioresource Technology 83(1): 37-46
- (2002b). Energy Production from Biomass (part 2): Conversion Technologies. Bioresource Technology 83(1): 47-54
Meyer, T. J. (1989). Chemical Approaches to Artificial Photosynthesis. Accounts of Chemical Research 22(5): 163-170
Milliron, D. J., S. M. Hughes, S.M. H., Cui S. M., C. S.M., Y. Cui, Y. C. (2004). Colloidal Nanocrystal Heterostructures with Linear and Branched Topology. Nature 430(6996): 190-195
Minh, N. Q. (1993). Ceramic Fuel-Cells. Journal of the American Ceramic Society 76(3): 563-588
Mitchell, J. F. B. (1989). The “Greenhouse” Effect and Climate Change. Reviews of Geophysics 27(1): 115-139
Montenegro, A., V. Brovkin, V. B., Eby V., E. V. (2007). Long Term Fate of Anthropogenic Carbon. Geophysical Research Letters 34: L19707
Mori, D., H. Kobayashi, H. K., Shikano H., S. H., M. Shikano, M. S., Nitani M. (2009). Bulk and Surface Structure Investigation for the Positive Electrodes of Degraded Lithium-Ion Cell after Storage Test Using X-Ray Absorption Near-Edge Structure Measurement. Journal of Power Sources 189(1): 676-680
Morison, J. I. L., D. W. Lawlor (1999). Interactions Between Increasing CO. Plant Cell and Environment 22(6): 659-682
Muller, A. (2009). Sustainable Agriculture and the Production of Biomass for Energy Use. Climatic Change 94(3–4): 319-331
Muradov, N. Z., T. N. Veziroglu (2008). “Green” Path from Fossil-based to Hydrogen Economy: An Overview of Carbon-Neutral Technologies. International Journal of Hydrogen Energy 33(23): 6804-6839
Music, S., S. Popovic, S. P., Maljkovic S., M. S. (2002). Influence of Synthesis Procedure on the Formation of RuO. Materials Letters 56(5): 806-811
Nakamura, J., Y. Choi, Y. C. (2003). On the Issue of the Active Site and the Role of ZnO in Cu/ZnO Methanol Synthesis Catalysts. Topics in Catalysis 22(3–4): 277-285
Nath, K., D. Das (2007). Production and Storage of Hydrogen: Present Scenario and Future Perspective. Journal of Scientific and Industrial Research 66: 701-709
Ni, M., M. K. H. Leung, M.K.H. L., Leung M. K. H. (2007). A Review and Recent Developments in Photocatalytic Water-splitting Using TiO. Renewable and Sustainable Energy Reviews 11(3): 401-425
Norskov, J. K., T. Bligaard, T. B., Logadottir T., L. T., A. Logadottir, A. L., Bahn A., B. A., S. Bahn, S. B., Hansen S., H. S. (2002). Universality in Heterogeneous Catalysis. Journal of Catalysis 209(2): 275-278
Olah, G. A. (2005). Beyond Oil and Gas: The Methanol Economy. Angewandte Chemie–International Edition 44(18): 2636-2639
Olah, G. A., A. Goeppert, A. G. (2009). Chemical Recycling of Carbon Dioxide to Methanol and Dimethyl Ether: From Greenhouse Gas to Renewable, Environmentally Carbon Neutral Fuels and Synthetic Hydrocarbons. Journal of Organic Chemistry 74(2): 487-498
Osterloh, F. E. (2008). Inorganic Materials as Catalysts for Photochemical Splitting of Water. Chemistry of Materials 20(1): 35-54
Padhi, A. K., K. S. Nanjundaswamy, K.S. N. (1997). Phospho-Olivines as Positive-Electrode Materials for Rechargeable Lithium Batteries. Journal of the Electrochemical Society 144(4): 1188-1194
Paraknowitsch, J. P., A. Thomas, A. T. (2009). Carbon Colloids Prepared by Hydrothermal Carbonization as Efficient Fuel for Indirect Carbon Fuel Cells. Chemistry of Materials 21(7): 1170-1172
Parmesan, C. (2006). Ecological and Evolutionary Responses to Recent Climate Change. Annual Review of Ecology Evolution and Systematics 37: 637-669
Parmesan, C., G. Yohe (2003). A Globally Coherent Fingerprint of Climate Change Impacts Across Natural Systems. Nature 421(6918): 37-42
Pasero, D., N. Reeves, N. R., Pralong N. (2008). Oxygen Nonstoichiometry and Phase Transitions in LiMn. Journal of the Electrochemical Society 155(4): A282-A291
Paustian, K., J. Six, J. S., Elliott J. (2000). Management Options for Reducing CO. Biogeochemistry 48(1): 147-163
Poschl, U. (2004). Interactive Journal Concept for Improved Scientific Publishing and Quality Assurance. Learned Publishing 17: 105-113
Ragauskas, A. J., C. K. Williams, C.K. W., Davison C. K., D. C.K., B. H. Davison, B.H. D., Britovsek B. H., B. B.H., G. Britovsek, G. B., Cairney G., C. G., J. Cairney (2006). The Path Forward for Biofuels and Biomaterials. Science 311: 484-489
Ramachandra, T. V. (2009). RIEP: Regional Integrated Energy Plan. Renewable and Sustainable Energy Reviews 13(2): 285-317
Raudaskoski, R., E. Turpeinen, E. T., Lenkkeri E., L. E. (2009). Catalytic Activation of CO. Catalysis Today 144(3–4): 318-323
Rossmeisl, J., K. Dimitrievski, K. D., Siegbahn K. (2007). Comparing Electrochemical and Biological Water Splitting. Journal of Physical Chemistry C 111(51): 18821-18823
Rossmeisl, J., Z. W. Qu, Z.W. Q., Zhu Z. W., Z. Z.W. (2007). Electrolysis of Water on Oxide Surfaces. Journal of Electroanalytical Chemistry 607(1–2): 83-89
Salem, R. R. (2008). Theory of the Electrolysis of Water. Protection of Metals 44(2): 120-125
Salmeron, M., R. Schlögl (2008). Ambient Pressure Photoelectron Spectroscopy: A New Tool for Surface Science and Nanotechnology. Surface Science Reports 63(4): 169-199
Schlapbach, L., A. Zuttel (2001). Hydrogen-Storage Materials for Mobile Applications. Nature 414(6861): 353-358
Schlögl, R. (2003). Catalytic Synthesis of Ammonia: A “Never-Ending Story”?. Angewandte Chemie International Edition 42(18): 2004-2008
Schlögl, R., T. Velden (2005). Open Access and the Berlin Declaration: The MPG Strategy. Abstracts of Papers of the American Chemical Society 229: 037-CINF
Schobert, H. H., C. Song (2002). Chemicals and Materials from Coal in the 21st Century. Fuel 81(1): 15-32
Schreiner, P. R. (2003). Metal-Free Organocatalysis through Explicit Hydrogen Bonding Interactions. Chemical Society Reviews 32: 289-296
Shaahid, S. M., I. El-Amin (2009). Techno-Economic Evaluation of Off-Grid Hybrid Photovoltaic-Diesel-Battery Power Systems for Rural Electrification in Saudi Arabia: A Way Forward for Sustainable Development. Renewable and Sustainable Energy Reviews 13(3): 625-633
Silva, N. F., Rosa da, R. d. (2005). The Utilization of Wind Energy in the Brazilian Electric Sector's Expansion. Renewable & Sustainable Energy Reviews 9: 289-309
Singhal, S. C. (2000). Advances in Solid Oxide Fuel Cell Technology. Solid State Ionics 135(1–4): 305-313
Sloczynski, J., R. Grabowski, R. G., Kozlowska R., K. R., A. Kozlowska, A. K. (2004). Catalytic Activity of the M/(3ZnO·ZrO. Applied Catalysis A: General 278(1): 11-23
Smeets, E. M. W., A. P. C. Faaij, A.P.C. F., Lewandowski A. P. C. (2007). A Bottom-Up Assessment and Review of Global Bio-Energy Potentials to 2050. Progress in Energy and Combustion Science 33(1): 56-106
Solomon, S. D., M. Quin, M. Q., Manning M., M. M. (2007). Climate Change 2007: The Physical Science Basis. Cambridge: Cambridge University Press.
Song, C. S., H. H. Schobert (1996). Non-Fuel Uses of Coals and Synthesis of Chemicals and Materials. Fuel 75(6): 724-736
Sorensen, R. Z., L. J. E. Nielsen, L.J.E. N., Jensen L. J. E., J. L.J.E., S. Jensen, S. J. (2005). Catalytic Ammonia Decomposition: Miniaturized Production of CO. Catalysis Communications 6(3): 229-232
Steele, B. C. H., A. Heinzel (2001). Materials for Fuel-Cell Technologies. Nature 414(6861): 345-352
Steinfeld, A. (2005). Solar Thermochemical Production of Hydrogen: A Review. Solar Energy 78(5): 603-615
Tang, Q. L., Q. J. Hong, Q.J. H. (2009). CO. Journal of Catalysis 263(1): 114-122
Tarascon, J. M., M. Armand (2001). Issues and Challenges Facing Rechargeable Lithium Batteries. Nature 414(6861): 359-367
Titirici, M. M., M. Antonietti, M. A. (2008). Hydrothermal Carbon from Biomass: A Comparison of the Local Structure from Poly- to Monosaccharides and Pentoses/Hexoses. Green Chemistry 10(11): 1204-1212
Titirici, M. M., A. Thomas, A. T., Yu A., Y. A. (2007). A Direct Synthesis of Mesoporous Carbons with Bicontinuous Pore Morphology from Crude Plant Material by Hydrothermal Carbonization. Chemistry of Materials 19(17): 4205-4212
Tributsch, H. (2008). Photovoltaic Hydrogen Generation. International Journal of Hydrogen Energy 33(21): 5911-5930
Turner, J., G. Sverdrup, G. S., Mann G., M. G., M. K. Mann, M.K. M., Maness M. K. (2008). Renewable Hydrogen Production. International Journal of Energy Research 32(5): 379-407
Umeyama, T., H. Imahori (2008). Carbon Nanotube-modified Electrodes for Solar Energy Conversion. Energy and Environmental Science 1(1): 120-133
Van den Heever, S. A., I. E. Grossmann (1999). Disjunctive Multiperiod Optimization Methods for Design and Planning of Chemical Process Systems. Computers and Chemical Engineering 23(8): 1075-1095
Van Heek, K. H. (2000). Progress of Coal Science in the 20th Century. Fuel 79(1): 1-26
Van Hise, J. R. (2008). CO. Research Journal of Chemistry and Environment 12(2): 14-16
Velden, T., C. Lagoze (2009). Communicating Chemistry. Nature Chemistry 1: 673-678
Velterop, J. (2003). Should Scholarly Societies Embrace Open Access (or Is it the Kiss of Death)?. Learned Publishing 16: 167-169
Volkening, S., K. Bedurftig, K. B., Jacobi K., J. K. (1999). Dual-Path Mechanism for Catalytic Oxidation of Hydrogen on Platinum Surfaces. Physical Review Letters 83(13): 2672-2675
Voorspools, K. (2004). Sustainability of the Future: Rethinking the Fundamentals of Energy Research. Renewable and Sustainable Energy Reviews 8(6): 599-608
Wakihara, M. (2001). Recent Developments in Lithium Ion Batteries. Materials Science and Engineering: R: Reports 33(4): 109-134
Wang, L. F., R. T. Yang (2008). New Sorbents for Hydrogen Storage by Hydrogen Spillover: A Review. Energy and Environmental Science 1(2): 268-279
Wontcheu, J., W. Bensch, W. B., Wilkening W., W. W., M. Wilkening, M. W., Heitjans M., H. M. (2008). Tuning the Structural and Physical Properties of Cr. Journal of the American Chemical Society 130(1): 288-299
Woodhouse, M., B. A. Parkinson (2009). Combinatorial Approaches for the Identification and Optimization of Oxide Semiconductors for Efficient Solar Photoelectrolysis. Chemical Society Reviews 38(1): 197-210
Xu, X. D., J. A. Moulijn (1996). Mitigation of CO. Energy and Fuels 10(2): 305-325
Yagi, M., A. Syouji, A. S., Yamada A., Y. A., S. Yamada (2009). Molecular Catalysts for Water Oxidation toward Artificial Photosynthesis. Photochemical and Photobiological Sciences 8(2): 139-147
Yang, C., Z. Y. Ma, Z.Y. M., Zhao Z. Y., Z. Z.Y., N. Zhao (2006). Methanol Synthesis from CO. Catalysis Today 115(1–4): 222-227
Zachos, J., M. Pagani, M. P., Sloan M., S. M. (2001). Trends, Rhythms, and Aberrations in Global Climate 65 Ma to Present. Science 292(5517): 686-693
Zhang, J., Y. S. Hu, Y.S. H., Tessonnier Y. S., T. Y.S., J. P. Tessonnier, J.P. T. (2008). CNFs@CNTs: Superior Carbon for Electrochemical Energy Storage. Advanced Materials 20(8): 1450-1455
Zwart, R. W. R., H. Boerrigter (2005). High Efficiency Co-production of Synthetic Natural Gas (SNG) and Fischer−Tropsch (FT) Transportation Fuels from Biomass. Energy and Fuels 19(2): 591-597